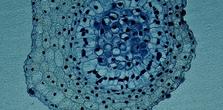
Circadian Rhythm Synchronization as a Strategy for Enhancing Healthspan and Preventing Metabolic Disorders
Circadian rhythms, regulated by the suprachiasmatic nucleus (SCN), orchestrate key physiological processes such as metabolism, hormonal release, and sleep-wake cycles. Disruptions to these rhythms—caused by factors like shift work, irregular sleep, or late-night eating—are linked to metabolic disorders, including obesity, diabetes, and cardiovascular disease. This review explores how the circadian clock regulates metabolic pathways, particularly AMPK, SIRT1, and mTOR, which influence glucose metabolism, fat storage, and cellular repair. External factors like light exposure, meal timing, and sleep consistency are essential for maintaining a robust circadian rhythm. Blue light disrupts melatonin production and metabolism, while red light may support better circadian alignment. Optimizing lifestyle habits in harmony with circadian rhythms may enhance metabolic health, promote longevity, and mitigate the risks of chronic diseases.
37 mins
By: Kristen Race, Shreshtha Jolly, Shriya Bakhshi
Introduction
Our bodies are finely tuned to the rhythms of the day, guided by an internal clock that affects far more than sleep. This biological timekeeper, known as the circadian rhythm, operates on a roughly 24-hour cycle and influences everything from waking up to processing food, regulating energy, and even how our brain functions. It controls hormonal fluctuations, body temperature, digestion, and cognitive abilities, and its influence extends into nearly every corner of our physiology. Disruptions to this rhythm, whether from late-night eating, irregular sleep schedules, or shift work, can have profound effects, contributing to metabolic disorders like diabetes, obesity, and cardiovascular disease.
In this review, we'll dive deep into the fundamentals of the circadian rhythm—how it works, why it's crucial for optimal metabolic health, and its wide-ranging effects on processes like fat storage, glucose metabolism, and even cognitive function. We'll also explore the role of circadian amplitude, the difference between robust and weak circadian cycles, and how maintaining a strong rhythm can protect against chronic diseases. Additionally, we'll break down the phases of the circadian cycle, from the cortisol surge in the morning that boosts alertness to the release of melatonin at night that prepares the body for rest. Along the way, we'll examine key factors influencing the circadian rhythm—light exposure, sleep patterns, and meal timing—and how aligning these daily habits with your internal clock can improve overall health and longevity.
This review also examines the connection between circadian rhythms and metabolic pathways, including its interaction with key regulators like AMPK, SIRT1, and mTOR. Understanding these connections shows why a well-synchronized circadian rhythm prevents metabolic imbalances, supports cognitive function, and promotes healthy aging. Whether you're seeking to optimize your daily habits or looking for a deeper understanding of the science behind the body's timekeeping system, this overview provides valuable insights into how your internal clock influences health and longevity.
Fundamentals of the Circadian Rhythm
The circadian rhythm is an internal biological clock that regulates the sleep-wake cycle and coordinates a range of physiological processes, operating on an approximately 24-hour cycle. Its influence extends beyond sleep, impacting hormonal release, body temperature, digestion, and cognitive function. To maintain optimal health and promote longevity, it is crucial that metabolic processes remain synchronized with the circadian clock. A growing body of research indicates that disruptions to this rhythm can lead to various metabolic and chronic diseases, while, conversely, proper alignment can enhance overall health [4]. Throughout this article, we will analyze how this misalignment leads to compromised metabolic function.
The circadian clock plays a crucial role in regulating metabolic pathways, particularly those involved in glucose and fat metabolism. This means that the body's ability to manage blood sugar levels, store or burn fat, and regulate energy is tightly controlled by this internal clock. Therefore, maintaining a synchronized circadian rhythm is directly linked to improved health outcomes, such as reduced risks of obesity, diabetes, and cardiovascular diseases [1].
Molecular Basis of Circadian Rhythms
Circadian rhythms are internally generated cycles that follow an approximately 24-hour period, even in the absence of external cues like light. These rhythms are encoded by molecular clocks found in nearly every cell of the body, orchestrating daily patterns of behavior and physiology. The body’s circadian system is organized in a hierarchical manner, with a master clock located in the suprachiasmatic nucleus (SCN) of the hypothalamus serving as the central regulator. This master clock coordinates the activity of peripheral clocks located throughout various tissues and organs, ensuring that internal biological processes stay in sync with the external environment.
The SCN consists of approximately 20,000 neurons that form a unified circadian network, making it the central hub of circadian regulation. Unlike peripheral clocks, the SCN receives direct input from the retina via light exposure. This light input is critical because it allows the SCN to synchronize the body’s internal timing with the solar day, ensuring that our biological processes align with the external light-dark cycle. In turn, the SCN relays timing signals to peripheral clocks through hormonal signals and other systemic cues, creating a coordinated system that governs functions like sleep-wake cycles, hormone secretion, and metabolism.
While both the SCN and peripheral clocks share a similar molecular architecture that generates circadian rhythms, they differ in how tightly their cells are interconnected. The SCN has a high degree of intercellular coupling, meaning the neurons within this region communicate closely to form a robust and unified clock that is resistant to disturbances. This high level of coordination ensures that the SCN maintains a consistent ~24-hour cycle, even when the body faces internal or external disruptions.
In contrast, peripheral clocks, located in tissues such as the liver, heart, and kidneys, are more susceptible to adjustment. While they receive cues from the SCN, their timing can also be influenced by local metabolic conditions, including fluctuations in body temperature and circulating hormones. This flexibility allows peripheral clocks to adapt to the specific needs and status of the tissue they regulate. For example, meal timing can shift the rhythms of metabolic organs like the liver and pancreas, reflecting changes in energy availability.
This network of clocks—with the SCN as the master regulator and peripheral clocks responding to both systemic and local signals—ensures that the body’s biological processes are coordinated with the external environment while allowing individual organs to fine-tune their timing based on specific physiological needs.
Consider the interaction between the SCN and the liver's peripheral clock. In the morning, exposure to natural light signals the SCN, which adjusts the body’s wakefulness and initiates metabolic processes to prepare for the day. The SCN communicates to peripheral clocks through signals like the release of cortisol—a hormone that peaks in the morning to help mobilize energy. This signal reaches the liver, which begins to adjust its own circadian rhythm to optimize glucose metabolism and ensure that the body has the energy it needs throughout the day.
At the same time, peripheral clocks in the liver also respond to local cues like meal timing. For example, when you eat breakfast, the liver clock receives signals to metabolize food, synthesizing proteins, and processing glucose. However, the liver clock’s activity is still influenced by the SCN’s overarching cues, ensuring that digestion and energy storage align with the body’s larger metabolic needs as dictated by the external light-dark cycle.
In this way, the master clock (SCN) provides the general timing and coordination with the external world, while the peripheral clocks, like those in the liver, fine-tune local processes to meet the specific demands of the body’s immediate physiological state. This coordinated effort ensures that biological processes like energy balance, hormone release, and metabolism are in sync, optimizing health and function.
The Role of Circadian Amplitude and its Relationship to the Master Clock
The circadian rhythm’s functionality is often described in terms of circadian amplitude, which refers to the difference between the peak of a circadian oscillation and its baseline or equilibrium state. A higher amplitude indicates stronger fluctuations between high and low points, reflecting a more robust central circadian clock, primarily governed by the SCN. This robustness is crucial for ensuring that the body’s internal clocks, including both the master clock in the SCN and the peripheral clocks in various tissues, remain well-synchronized with each other and with external environmental cues, like the solar day. [5]
A high circadian amplitude suggests better synchronization and regulation of key physiological processes such as metabolism, hormone secretion, and energy balance. When the SCN, acting as the body’s master clock, sends strong and consistent signals to peripheral clocks, the body operates efficiently. This coordinated timing helps regulate everything from sleep-wake cycles to the body’s ability to process glucose, manage fat storage, and maintain overall energy balance. In turn, a higher amplitude translates to improved health outcomes and greater resilience against disruptions, whether they are internal (like metabolic changes) or external (like light exposure at night). [6]
Conversely, a low-amplitude circadian rhythm, characterized by weaker and less distinct oscillations, can lead to misalignment between the master clock in the SCN and peripheral clocks. This desynchronization disrupts critical metabolic functions, impairing the body’s ability to regulate hormonal cycles, maintain proper metabolic pathways, and balance energy efficiently. A weakened circadian amplitude has been linked to a host of chronic health issues, including depression, diabetes, cardiovascular disease, and cancer. [6]
Factors like shift work, irregular meal timing, or excessive light exposure at night can further weaken circadian amplitude. These disruptions reduce the SCN’s ability to send strong, consistent signals to peripheral clocks, leading to dysregulated metabolic pathways. The result can be insulin resistance, elevated blood glucose levels, and fat accumulation, all of which contribute to chronic metabolic diseases.
In contrast, strengthening circadian amplitude by aligning eating habits, physical activity, and sleep patterns with the body's natural circadian rhythm can improve the synchronization between the master clock and peripheral clocks. This enhances metabolic efficiency, improves sleep quality, and supports overall longevity.
Phases of Circadian Rhythm and the Role of the Master Clock
The circadian rhythm operates through distinct phases, each of which governs specific physiological processes essential for health and performance. These phases are tightly regulated by the master clock in the SCN, which coordinates signals to peripheral clocks throughout the body. Understanding these phases can help optimize daily activities by aligning them with your body’s internal clock for enhanced well-being. [1, 2, 4]
Morning Phase (Wakefulness)
Cortisol Surge: Upon waking, cortisol levels experience a sharp rise through the cortisol awakening response (CAR). Cortisol acts as the body’s internal alarm clock, boosting alertness, regulating blood pressure, and kick-starting energy levels for the day. The SCN triggers this cortisol surge to ensure that the body’s metabolic and hormonal processes align with daylight hours. Cortisol also plays a role in glucose metabolism, preparing the body to efficiently use energy after the overnight fast.
Increased Body Temperature: In the morning, body temperature gradually rises, which is regulated by the SCN. This increase supports wakefulness and cognitive function and is crucial for optimizing physical performance and mental alertness as the day begins.
Peak in Cognitive Function: Cognitive abilities such as problem-solving, memory recall, and concentration typically peak in the late morning, aligning with the SCN’s coordination of neural and metabolic processes. This is an ideal time for mentally demanding tasks.
Daytime Phase (Peak Activity)
High Alertness and Performance: From late morning to early afternoon, the body is at its peak in terms of alertness, physical performance, and cognitive function. The SCN synchronizes these rhythms with the external light-dark cycle, making this the best time for exercise and complex cognitive tasks.
Decreasing Cortisol Levels: After its morning peak, cortisol levels gradually decline throughout the day, signaling the body to transition from high-energy activities to more relaxed states. This tapering reflects the SCN's role in managing energy balance across different times of day.
Afternoon Dip (Post-Lunch Dip)
Mild Drop in Alertness: Many people experience a natural dip in energy in the early afternoon, often referred to as the "post-lunch dip." This is driven by circadian fluctuations, where the peripheral clocks in tissues like the liver and digestive system may slow metabolic activity, contributing to the dip. Blood sugar levels after eating can also play a role.
Body Temperature Peak: Despite the dip in alertness, body temperature reaches its highest point in the early afternoon, supporting continued productivity and physical performance. The SCN continues to regulate this phase, coordinating thermal regulation and activity levels.
Evening Phase (Preparation for Sleep)
Melatonin Release: As daylight fades, the SCN signals the pineal gland to secrete melatonin, the hormone that prepares the body for sleep. Melatonin levels rise in response to darkness, aligning the body’s internal timing with the external environment. This transition is crucial for synchronizing the master clock with peripheral clocks in tissues involved in metabolism and energy regulation.
Thyroid-Stimulating Hormone (TSH): TSH regulates the production of thyroid hormones, which are essential for controlling metabolism, energy levels, and overall bodily function. As melatonin increases in the evening, it suppresses TSH, helping reduce metabolic activity and prepare the body for rest.
Prolactin: Melatonin stimulates prolactin secretion during the night, with prolactin levels typically peaking during sleep. While prolactin is primarily involved in lactation, it also plays important roles in immune regulation and reproductive health, contributing to nighttime bodily restoration and immune function.
Leptin: Melatonin helps enhance leptin sensitivity, which signals to the brain when to stop eating. This process regulates appetite and promotes metabolic efficiency during sleep. Leptin levels typically rise overnight, aiding in energy balance and preventing late-night hunger, contributing to overall weight management.
Growth Hormone (GH): Melatonin plays a significant role in triggering the release of growth hormone, which reaches its highest levels during deep sleep. GH is essential for stimulating growth, repairing tissues, and maintaining muscle and bone health. The synchronization of melatonin and GH release underscores the importance of deep sleep for physical recovery and long-term health.
Decreased Body Temperature: As melatonin levels rise, body temperature drops, preparing the body for rest. This cooling effect helps facilitate deeper sleep and improves overall sleep quality.
Key Factors Influencing the Circadian Rhythm
To effectively anchor your circadian rhythm, three key variables are at your disposal: light exposure, consistent sleep-wake times, and meal timing.
Light is the most powerful external cue, or zeitgeber, for regulating the circadian rhythm. Morning light exposure helps reset and synchronize your internal clock, promoting wakefulness and alertness, while reducing light exposure in the evening stimulates melatonin release, preparing the body for sleep.
Consistency in sleep and wake times is equally important. Irregular sleep patterns—such as staying up late or waking at varying times—can disrupt the circadian rhythm, leading to poor sleep quality and affecting overall health.
Similarly, regular meal timing plays a significant role, as the body's internal clock governs digestion and metabolism. Eating at consistent times helps synchronize these metabolic processes, promoting better health and energy balance. [6]
Optimizing Health by Aligning with the Circadian Rhythm
Why is aligning the circadian rhythm so critical to healthspan? The circadian rhythm’s importance extends beyond regulating sleep—it is fundamental to our metabolic health. Many key metabolic pathways, including how we metabolize energy at the cellular level, are deeply influenced by our internal clock.
By aligning daily habits—such as eating, exercising, and sleeping—with the natural rhythm of our internal clock, we can enhance the body’s ability to regulate glucose, manage fat storage, and maintain muscle and bone health. Conversely, disruptions to this rhythm, such as late-night eating or exposure to artificial light after dark, can desynchronize these vital processes. This misalignment increases the risk of metabolic disorders, impairs sleep quality, and can reduce longevity. [6]
Let’s explore how circadian misalignments manifest in key measures of health span, starting with cognitive function.
Circadian Rhythm and Cognitive Function
In a study titled "Associations between Circadian Alignment and Cognitive Functioning in a Nationally Representative Sample of Older Adults", published in the prestigious journal Nature, Leahy et al. (2024) explored the connection between circadian alignment and cognitive decline in adults aged 60 and above. The study used data from the National Health and Nutrition Examination Survey (NHANES) to examine how well an individual’s internal biological clock synchronized with external cues like daylight and darkness. This alignment, regulated by the SCN, is essential not only for maintaining healthy sleep-wake cycles but also for supporting cognitive processes. Although cognitive function—including memory, attention, problem-solving, and processing speed—naturally declines with age, the study suggests that disruptions in circadian rhythms can accelerate this deterioration. [3]
To assess circadian alignment, the researchers used actigraphy, a method that tracks movement patterns via a wrist-worn device to determine periods of activity and rest. This data helped the researchers understand how closely participants’ sleep-wake patterns corresponded with the natural light-dark cycle governed by the SCN. Two key metrics were derived from the actigraphy data:
Phasor magnitude: A measure of how strongly physical activity aligns with the light-dark cycle, reflecting the robustness of circadian amplitude.
Phasor angle: The timing difference between physical activity and exposure to light, indicating the level of synchronization between internal and external cues.
The study used multiple linear regression models to analyze the relationships between circadian metrics like phasor magnitude and phasor angle and participants' cognitive test scores. Linear regression models are essential tools for understanding how one or more independent variables (circadian alignment metrics) influence a dependent variable (cognitive performance). By using this method, the researchers determined the strength and direction of the relationship between circadian alignment and cognitive function. Importantly, the analysis controlled for potential confounders such as age, gender, and educational background, ensuring that the observed effects were specifically due to circadian alignment.
The findings revealed that participants with a lower phasor magnitude—indicating weaker circadian alignment—performed significantly worse on cognitive tasks, exhibiting slower processing speed and reduced working memory. This highlights the crucial role of a robust circadian rhythm, regulated by the SCN, in maintaining cognitive function. Poor circadian alignment can lead to miscommunication between the SCN and peripheral clocks, impairing cognitive processes and leading to faster cognitive decline.
The results emphasize the broader implications of circadian misalignment, showing that it impacts more than just sleep—it can impair cognitive performance, particularly in older adults. As the SCN’s coordination with external environmental cues breaks down, sleep and cognitive functions, such as memory, attention, and processing speed, deteriorate.
This research underscores the importance of interventions that can improve circadian alignment. By aligning the body’s internal clock with external cues, particularly through managing light exposure and maintaining consistent sleep-wake cycles, it may be possible to mitigate the effects of aging on the brain and overall health. This alignment could support longevity and well-being by preserving both cognitive function and physiological balance.
The Interaction Between AMPK and the Circadian Rhythm
One of the key metabolic pathways that interacts with our circadian rhythm is the AMPK signaling pathway. AMPK, or AMP-activated protein kinase, serves as a crucial energy sensor in the body. It becomes activated in response to low-energy states—such as fasting, exercise, or caloric restriction—when cellular energy levels drop, signaled by a rise in AMP and a decrease in ATP. By detecting these low energy levels, AMPK initiates processes that restore energy balance and promote metabolic health.
For example, in the morning, as the body transitions from its rest phase to its active phase, AMPK activity is relatively low because food intake raises energy levels. During this time, glucose uptake and fatty acid oxidation are synchronized with the active phase to meet the increased demand for energy. This aligns with the suprachiasmatic nucleus (SCN), which signals the body's internal clocks to ensure energy is readily available after fasting overnight. The degradation of CRY1 by AMPK ensures that these metabolic processes are properly aligned with the circadian rhythm.
As the day progresses to the afternoon, when the body is at its peak activity, AMPK continues to monitor nutrient availability. After meals, energy is abundant, so AMPK activity remains low, allowing for glucose metabolism to meet energy needs during physical and mental activity. The SCN, in conjunction with peripheral clocks, maintains this synchronization to maximize energy efficiency.
In the evening, as the body prepares for rest, AMPK activity increases again due to a decrease in food intake. This activation promotes fatty acid oxidation and suppresses energy-consuming processes like fat synthesis, helping the body conserve energy. AMPK signals to peripheral clocks, aligning metabolic processes with the circadian rhythm to reduce energy expenditure in preparation for the rest phase. During this time, CRY1 destabilization ensures that metabolic activity aligns with the transition to the night phase.
Finally, during the night, AMPK is highly active due to the fasting state that occurs during sleep. This ensures that the body conserves energy, shifting metabolism toward fat oxidation. The SCN communicates with peripheral clocks to reduce energy expenditure and focus on repair and recovery, maintaining synchronization between the circadian clock and metabolic processes.
In addition to these phase-specific roles, AMPK stimulates catabolic processes that generate energy, such as fatty acid oxidation and glucose uptake, while suppressing anabolic processes like fatty acid synthesis and protein synthesis. This balance ensures that energy is efficiently utilized and conserved during times of energy shortage, such as fasting or exercise. [7, 22]
Moreover, AMPK directly influences the circadian clock by triggering the phosphorylation and degradation of cryptochrome-1 (CRY1), a core clock protein crucial in regulating the body’s internal timing. By destabilizing CRY1 during low-energy states, AMPK acts as a molecular bridge, linking the body’s metabolic status to the circadian clock. This synchronization ensures that energy utilization and metabolic processes are aligned with the appropriate times of day, optimizing overall energy efficiency [7, 22].
Several factors can activate AMPK, each contributing to improved metabolic health and longevity:
Dietary Restriction: Caloric restriction (reducing calorie intake without causing malnutrition) is one of the most well-researched methods of activating AMPK. By lowering the body's energy intake, caloric restriction reduces cell ATP levels, triggering AMPK activation. This leads to an increase in fat breakdown (fatty acid oxidation) and a decrease in fat production (fatty acid synthesis). The activation of AMPK in this context improves insulin sensitivity, enhances energy metabolism, and may delay the onset of age-related diseases. [8]
Intermittent Fasting: Similar to dietary restriction, intermittent fasting involves cycling between periods of eating and fasting. During fasting periods, glucose and insulin levels drop, leading to a reduction in available energy, which activates AMPK. This activation promotes several metabolic benefits, including improved glucose regulation, enhanced fat burning, and reduced inflammation. Furthermore, intermittent fasting triggers autophagy, the body's way of clearing out damaged cells and regenerating new, healthy cells—another mechanism associated with longevity.
Physical Activity: Exercise is one of the most effective natural triggers for AMPK activation. During physical activity, muscles require more energy, causing ATP levels to drop and AMP levels to rise, which activates AMPK. This activation promotes glucose uptake into muscles, enhances fat oxidation for fuel, and improves overall energy efficiency. Regular exercise thus supports metabolic health and may protect against conditions like obesity, diabetes, and cardiovascular disease by continually activating AMPK and improving insulin sensitivity.
Pharmacological Interventions: Several medications and compounds have been shown to activate AMPK or modulate related pathways, offering potential therapeutic benefits for aging and metabolic diseases. One such compound is metformin, a widely used drug for managing type 2 diabetes. Metformin works partly by activating AMPK, which helps reduce liver glucose production, improves insulin sensitivity, and lowers blood sugar levels. By activating AMPK, metformin also mimics some of the metabolic benefits of caloric restriction, which has made it a focus of research on aging and longevity. [9]
Optimizing the interaction between AMPK and the circadian rhythm can significantly impact health, particularly for those looking to improve metabolic efficiency, energy balance, and overall well-being. This is specifically important when it comes to feeding schedule and scheduling sleep and recovery.
In terms of optimizing feeding schedule, by understanding AMPK's role in energy sensing and circadian rhythm regulation, you can implement time-restricted eating (TRE) or intermittent fasting strategies to enhance metabolic health. As we mentioned, AMPK is activated during fasting periods when energy levels are low, promoting fat oxidation and improving insulin sensitivity. Aligning food intake with the body's natural circadian rhythm—eating during daylight hours and fasting overnight—can further enhance the body’s ability to regulate glucose and manage fat storage efficiently [22]. This strategy helps synchronize metabolism with the SCN, optimizing energy use during active hours and promoting cellular repair during rest.
Additionally, understanding how AMPK activity increases during the night phase allows individuals to support their body’s recovery and repair processes through optimized sleep patterns. Melatonin release and the stabilization of energy balance at night ensure that the body shifts into fat oxidation and cellular repair. Prioritizing consistent sleep schedules, especially in line with natural light-dark cycles, can further enhance the synchronization of metabolic and circadian processes, improving overall sleep quality and promoting long-term health [22, 23].
Moreover, because AMPK links nutrient signals to the circadian clock, maintaining a healthy circadian rhythm—through regular sleep-wake cycles, timed meals, and exposure to natural light—can further optimize metabolic processes and support healthy aging. The interplay between AMPK activation and circadian alignment demonstrates the importance of synchronizing metabolic health with daily biological rhythms, reinforcing the body's natural ability to maintain balance and promote longevity. [9]
Circadian Rhythms and Metabolic Pathways
Apart from AMPK signaling, our circadian rhythms are intricately connected to several other metabolic pathways that synchronize the body's energy usage, storage, and overall metabolism with the circadian clock. This coordination is essential for maintaining metabolic health, ensuring that key processes like energy production, fat metabolism, and cell growth occur in harmony with the sleep-wake cycle.
The SIRT1 Pathway
One metabolic pathway closely tied to circadian rhythms is the Sirtuin 1 (SIRT1) pathway, a nuclear protein that plays a pivotal role in metabolism, DNA repair, inflammation, and oxidative stress. Acting as an energy sensor, SIRT1 responds to changes in cellular energy levels, making it a critical regulator of metabolic health. Due to its broad involvement in cellular processes, SIRT1 is a key target in efforts to promote longevity and combat age-related diseases. [10]
SIRT1 has a direct connection to the circadian clock, specifically with BMAL1, a core clock protein that regulates genes responsible for circadian rhythms. BMAL1 is crucial in maintaining metabolic homeostasis; its deficiency is linked to premature aging and reduced lifespan. This is due to BMAL1’s role in regulating the mTORC1 complex, a critical player in mTOR signaling. The mTOR pathway regulates cell growth, metabolism, and autophagy (the recycling of cellular components). However, as we age, mTORC1 can become overactive, contributing to age-related conditions like cancer, neurodegeneration, and insulin resistance. [11]
SIRT1 helps modulate the mTOR pathway by interacting with BMAL1, suppressing excessive mTORC1 activity and promoting autophagy. This regulation is crucial, as it ensures that mTOR signaling remains balanced, promoting repair processes, especially during periods of low energy, such as fasting. This process highlights the importance of aligning meal timing with the circadian rhythm. [12]
Crucially, SIRT1 also plays a direct role in regulating glucose and lipid metabolism, mitochondrial function, and the body’s resistance to stress. By modulating the circadian clock, SIRT1 helps synchronize these metabolic processes with the day-night cycle, ensuring that energy utilization aligns with periods of activity during the day and rest during the night. For example, during the active phase (daytime), SIRT1 promotes glucose utilization and fat oxidation, optimizing energy availability. In contrast, during the rest phase (nighttime), SIRT1 supports energy storage and tissue repair.
By scheduling meals within the appropriate hours of the day-night cycle, individuals can optimize the activity of SIRT1 and other circadian-regulated pathways like mTOR, ensuring that energy consumption, metabolism, and cellular repair processes occur in sync with the body's natural rhythms. This approach can help prevent metabolic dysfunction and enhance longevity by allowing the body to efficiently manage energy resources, avoid unnecessary growth signals at night, and focus on cellular maintenance.
Circadian Control of the mTOR Pathway
In addition to its indirect regulation by SIRT1, the circadian clock also directly influences the mTOR pathway. mTOR signaling plays a key role in regulating protein synthesis, cell growth, and metabolism. The circadian clock controls the timing of these processes by promoting mTOR activity during periods when nutrients are more readily available—typically during the daytime. This alignment ensures that metabolic processes such as protein synthesis, lipid metabolism, and autophagy occur when the body has the necessary energy and nutrients to support them. [12]
For example, during the day, when food intake is more frequent, circadian rhythms promote mTOR signaling to stimulate protein synthesis, which is crucial for muscle maintenance and repair. Conversely, during the night, when energy intake is lower, the body shifts focus toward autophagy, recycling cellular components to conserve energy and support cellular maintenance. [12]
This circadian regulation of mTOR ensures that energy-consuming processes, like protein synthesis and cell growth, are tightly aligned with feeding-fasting cycles and overall energy availability. This promotes efficient energy use and helps prevent metabolic disorders resulting from the misalignment of circadian rhythms, such as obesity and insulin resistance. [12]
The PPAR Pathway
The circadian clock also exerts regulatory control over the Peroxisome Proliferator-Activated Receptor (PPAR) pathway, which plays a crucial role in how the body uses and stores fat. There are three main types of PPARs: PPARα, PPARγ, and PPARβ/Δ. These receptors regulate the expression of genes involved in lipid metabolism, aligning fat storage and utilization with feeding times. [11, 12]
PPARα is primarily expressed in tissues that rely heavily on fatty acid oxidation, such as the circadian clock, which regulates the liver and muscles and its activity. PPARα helps regulate lipid metabolism by promoting the breakdown of fats for energy during periods of fasting. In contrast, PPARγ is more involved in fat storage and insulin sensitivity. Its activity also follows a circadian rhythm, ensuring that fat storage is promoted during feeding times and that insulin sensitivity is optimized to manage blood glucose levels. [11, 12]
By aligning the activity of PPARs with feeding-fasting cycles, the circadian clock ensures efficient energy use and storage, promoting metabolic flexibility and reducing the risk of metabolic disorders.
Insulin Signaling and Glucocorticoids
Insulin signaling is another pathway tightly regulated by the circadian clock. Insulin is essential for glucose uptake and energy storage, and the sensitivity of tissues to insulin varies throughout the day. Typically, insulin sensitivity peaks during the body's active phase (daytime), allowing glucose to be efficiently absorbed and stored after meals. During the rest phase (nighttime), the body shifts from glucose metabolism to fat oxidation, conserving glucose for times of higher activity. This circadian modulation of insulin sensitivity helps synchronize glucose metabolism with feeding times, optimizing energy storage during the day and reducing the risk of metabolic disorders like type 2 diabetes.
The circadian clock also regulates the release of glucocorticoids, such as cortisol, which play critical roles in energy metabolism. Cortisol levels follow a circadian rhythm, peaking in the early morning and gradually declining throughout the day. This rhythm helps regulate glucose production (via gluconeogenesis) and fat breakdown (lipolysis), ensuring that energy is mobilized efficiently during the active phase and conserved during rest. [11, 12]
NAD+ and Coenzyme Regulation
Lastly, the circadian clock regulates certain coenzymes, such as NAD+, which play critical roles in metabolic processes. NAD+ is essential for the activity of SIRT1 and other NAD+-dependent enzymes that regulate the circadian clock and cellular energy metabolism. NAD+ participates in key processes such as glycolysis (breaking down glucose for energy), oxidative phosphorylation (producing ATP from nutrients), and fatty acid oxidation. Circadian regulation of NAD+ ensures that these processes are aligned with the body's energy demands throughout the day-night cycle, optimizing energy production during periods of activity and conserving resources during rest. [11, 12]
Risk Factors for Dysregulation of the Circadian Rhythm
Circadian rhythms play a fundamental role in regulating various metabolic pathways. By overseeing this complex signaling network, the circadian clock ensures that energy availability, storage, and expenditure are finely tuned to our natural rhythms, promoting overall metabolic health and longevity. However, disruptions to the circadian clock—such as irregular sleep patterns, shift work, or late-night eating—can impair these regulatory mechanisms, leading to metabolic imbalances and increasing the risk of diseases such as obesity, diabetes, and cardiovascular disorders. [13]
As people age, the strength and amplitude of circadian rhythms naturally weaken, increasing susceptibility to circadian misalignment, sleep disorders, and metabolic issues. This weakening of circadian regulation is often linked to changes in the suprachiasmatic nucleus (SCN) function, the brain's master clock, which governs the body's sleep-wake cycles and other circadian rhythms. With age, the SCN becomes less responsive to external cues like light, reducing synchronization between the internal body clock and environmental signals.
Irregular Sleep Patterns are one of the primary contributors to circadian clock dysregulation. Shift work, particularly night or rotating shifts, severely disrupts the natural sleep-wake cycle by misaligning the internal circadian clock with the external environment. Jet lag and inconsistent sleep schedules can also disturb the circadian rhythm, leading to poor sleep quality, fatigue, and impaired cognitive and metabolic functioning.
Exposure to bright screens—from devices such as computers, smartphones, and televisions—especially before bedtime further interferes with circadian regulation. The blue light emitted by these devices inhibits melatonin production, a hormone critical for initiating sleep. This suppression delays sleep onset and disrupts the natural circadian rhythm. Moreover, inadequate exposure to natural sunlight, particularly in the morning, can weaken the body's signals that regulate the circadian clock, leading to increased risks of circadian misalignment. [13]
Morning sunlight, shortly after sunrise, is therefore particularly beneficial for circadian rhythm regulation. If you wake before dawn, using bright artificial lights indoors can serve as a temporary measure, but these lights are less effective than natural sunlight. Once the sun rises, you must spend time outside and expose yourself to natural light, even on overcast days. On especially cloudy days, spending more time outdoors can compensate for lower light intensity, as light exposure's duration and strength play critical roles in adjusting and maintaining circadian rhythm. [13]
Red Light & Blue Light Effects on Circadian Rhythms and Glucose Metabolism
An important area of interest in circadian health research involves understanding the effects of red and blue light exposure on circadian rhythm regulation and its broader impact on glucose metabolism. Blue light, often emitted by electronic devices, inhibits melatonin production, delaying sleep onset and disrupting circadian rhythms. In contrast, red light, which is less disruptive to the circadian system, may support healthier circadian rhythms. By exploring the relationship between different types of light exposure, circadian function, and metabolic health, we can gain insights into optimizing our environments and behaviors for overall well-being. [14]
Visible light is a small part of the electromagnetic spectrum, ranging from radio waves to gamma rays. Within the visible light spectrum, blue light has a shorter wavelength (approximately 450–495 nm), while red light has a longer wavelength (around 620–750 nm), associated with warmer tones. Blue light, in particular, has been shown to significantly impact circadian rhythms and metabolic processes. Exposure to blue light, especially at night, can disrupt the circadian rhythm, which is closely linked to glucose metabolism. [14]
Research by Czeisler et al. (2015) showed that disrupting circadian rhythms can lead to decreased insulin sensitivity and impaired glucose tolerance, contributing to elevated blood glucose levels. Similarly, Cheung et al. (2016) found that evening exposure to blue-enriched light can increase glucose levels and impair glucose tolerance in healthy adults. These studies emphasize the importance of maintaining a properly functioning circadian clock, as excessive exposure to blue light in the evening can dysregulate circadian rhythms and impair glucose metabolism, increasing the risk of metabolic disorders like type 2 diabetes. [15]
Conversely, red light therapy has been studied for its potential to improve glucose metabolism and reduce insulin resistance. While red light does not directly affect circadian rhythms, it may enhance sleep quality, which is essential for the proper functioning of the circadian clock. Since the circadian clock is critical in regulating insulin signaling and glucose metabolism, red light therapy may indirectly improve these processes by supporting overall circadian health. [16]
Research supports this notion. De Freitas and Hamblin (2016) found evidence that red light can improve insulin sensitivity and glucose metabolism by reducing oxidative stress and inflammation. Another pilot study demonstrated that red light therapy reduced fasting blood glucose levels and improved glucose tolerance in patients with type 2 diabetes, suggesting that red light may have therapeutic potential for improving glucose regulation. [16]
These findings highlight the importance of managing light exposure, particularly limiting blue light at night, and exploring the potential benefits of red light for glucose metabolism. Optimizing light exposure, especially during critical times of the day, may protect circadian health and support metabolic function.
Far-Reaching Effects of Circadian Rhythm Disruption on Physical and Mental Health
A dysfunctional circadian rhythm can lead to a wide range of symptoms, affecting both physical and mental health. These symptoms can vary depending on the severity and duration of the circadian disruption. In addition to metabolic dysregulation and cognitive impairments, circadian rhythm disruptions can impact multiple organ systems throughout the body. [1, 2, 3]
Sleep disturbances are common, including insomnia, where individuals have difficulty falling asleep, staying asleep, or waking up too early. Many also experience excessive daytime sleepiness, even after a full night's rest, and irregular sleep patterns, characterized by inconsistent bedtimes and wake-up times. Mood disturbances are another significant consequence, with symptoms such as irritability, heightened anxiety, and even depression leading to persistent feelings of sadness or hopelessness. [1, 2, 3]
Physical symptoms of circadian dysfunction include fatigue, which persists despite adequate rest, digestive issues like indigestion and bloating, and frequent headaches or migraines, often linked to poor sleep quality. Hormonal imbalances can also occur, manifesting as disrupted menstrual cycles in women or reduced libido and sexual performance issues in both men and women. Cardiovascular issues may arise, including increased blood pressure and heart palpitations, often tied to stress or sleep problems. [1, 2, 3]
Impaired immune function is another serious consequence, as circadian disruptions can increase susceptibility to infections, prolong recovery times, and contribute to chronic low-grade inflammation, exacerbating various health conditions. Emotional dysregulation may present as mood swings and an increased sensitivity to stress, making it difficult for individuals to cope with normal stressors, leading to exaggerated emotional responses. [1, 2, 3]
If not properly managed, a disrupted circadian rhythm can raise the risk of chronic conditions such as heart disease, diabetes, obesity, and mental health disorders. Because circadian rhythms are closely linked to metabolism, any misalignment in the body's internal clock can have far-reaching effects on metabolic health, further contributing to these chronic health issues.[1, 2, 3]
Effects of Chronotype on Circadian Rhythm Function and Metabolic Health
A chronotype refers to an individual's natural preference for sleep and wake times, dictated by their internal biological clock. It determines whether someone is more alert and energetic in the morning (commonly called an "early bird"), in the evening ("night owl"), or falls somewhere in between (an "intermediate" or "neutral type"). Chronotypes influence sleep patterns, mood, and daily performance, and they can vary based on age, lifestyle, and genetic factors. [18]
Research has identified two primary chronotype categories linked to human genetic polymorphisms: early chronotypes and late chronotypes. Genetic polymorphisms are variations in DNA sequences that occur among individuals in a population, affecting traits like sleep-wake patterns and circadian rhythms. Individuals with a late chronotype often experience a misalignment between their internal clock and societal norms, which typically favor early waking hours. This misalignment can lead to various health issues. For example, multiple studies, including research by Nimitphong et al. (2018), have found that late chronotypes are associated with an increased risk of prediabetes and type 2 diabetes, primarily due to higher levels of inflammation. This inflammation can disrupt metabolic processes and contribute to insulin resistance. [18]
External factors such as shift work and social jetlag can exacerbate circadian misalignment, particularly for specific chronotypes, negatively impacting circadian rhythm functioning and metabolic health. Social jetlag occurs when an individual's internal circadian clock is disrupted by social obligations, leading to a mismatch between their preferred sleep patterns and societal demands. It is measured as the difference in hours between the midpoint of sleep on workdays and the midpoint on days without commitments. For example, a "night owl" might sleep from 10:00 pm to 6:00 am on workdays but from 1:00 am to 10:00 am on free days, creating a social jetlag of 3.5 hours (with the midpoint of sleep at 2:00 am on workdays and 5:30 am on free days). This accumulated misalignment throughout the week can cause short-term declines in performance and long-term metabolic impairments. [19]
A study by Koopman et al. (2017) explored the relationship between social jetlag and metabolic syndrome, as well as type 2 diabetes, in a population-based cohort. Social jetlag was calculated by comparing the midpoint of sleep on weekdays to that on weekends, and participants were grouped into three categories based on the magnitude of their social jetlag: no social jetlag (<1 hour), 1-2 hours of social jetlag, and >2 hours of social jetlag. The study found that individuals with greater social jetlag, particularly those younger than 61 years, had a two-fold increased risk of developing metabolic syndrome and diabetes or prediabetes. [20]
Overall, chronotype significantly affects circadian rhythm functioning and metabolic health. Late chronotypes, in particular, face heightened risks of misalignment and related health issues. Recognizing the impact of social jetlag and making lifestyle adjustments to align better with one's chronotype can help mitigate these risks and promote improved metabolic health across all chronotypes.
Gaps in Circadian Rhythm Research
One of the primary gaps in current research is the need for more understanding regarding the impact of dietary interventions on circadian rhythms. Various dietary strategies, such as dietary restriction (DR), calorie restriction (CR), intermittent fasting (IF), low-carbohydrate diets (low-carb), and ketogenic diets (KD), have been shown to provide significant health benefits. However, the specific effects of these diets on the circadian clock and their interplay with circadian rhythms remain largely unexplored. Understanding how these dietary interventions influence circadian rhythms is crucial for optimizing their health benefits and could lead to more tailored dietary recommendations for promoting longevity. [21]
Another significant gap is the heavy reliance on animal models, particularly mice, in much of the existing research on circadian rhythms and aging. While rodent studies have yielded valuable insights, they may only partially translate to human physiology due to fundamental differences between species. More human-based studies are necessary to draw more accurate conclusions about the relationship between circadian rhythms and longevity. These studies would help clarify how circadian rhythms affect various aspects of health and aging in diverse human populations.
Low compliance in clinical trials presents another challenge, particularly in studies involving dietary interventions and lifestyle changes. Inadequate adherence can hinder the collection of reliable data. For example, in the Koopman et al. (2017) study, fewer than 0.4% of participants experienced social jetlag of more than 3 hours, limiting the ability to evaluate the effects of extreme circadian disruptions on metabolic functioning. Lack of adherence complicates the ability to assess the long-term effects of circadian rhythms on aging and longevity. Improving compliance in clinical trials is essential for gathering accurate data that can inform future health strategies.
An underexplored area in circadian research is how an individual's circadian clock can be leveraged to enhance the efficacy of existing anti-aging pharmacological interventions. While a well-functioning circadian clock independently promotes longevity, it may also help dictate the timing and dosages of anti-aging drug regimens for optimal outcomes. Exploring how circadian rhythms influence drug efficacy could lead to more effective treatment strategies for age-related diseases. Timing and dosage that align with an individual's circadian clock could enhance drug efficacy and improve health outcomes for older adults. Genome-wide studies suggest that the targets of over 80% of FDA-approved drugs exhibit activity patterns that follow circadian rhythms. Therefore, matching treatment regimens to circadian schedules is crucial for optimizing drug-mediated aging and longevity outcomes. [21]
Conclusion:
The circadian rhythm is far more than just a regulator of sleep—it is an essential system that affects our entire physiology, from metabolism to cognitive function. This review has explored how this internal clock governs processes like fat and glucose metabolism, body temperature, and hormone release. Maintaining a high-amplitude circadian rhythm ensures that these functions operate optimally, promoting overall health and reducing the risk of chronic diseases like obesity, diabetes, and cardiovascular issues.
By understanding the phases of the circadian cycle and aligning our habits—such as eating, exercising, and sleeping—with these natural rhythms, we can enhance metabolic efficiency, improve cognitive function, and support healthy aging. The interplay between circadian rhythm and key metabolic regulators like AMPK and SIRT1 further emphasizes this system's critical role in balancing energy and maintaining cellular health.
As research continues to illuminate the connections between circadian rhythm and long-term health, optimizing our daily routines to align with our internal clock becomes a powerful strategy for promoting longevity and reducing the risk of disease. By respecting and nurturing this natural cycle, we give our bodies the tools to function at their best.
TAKE HOME POINTS
Circadian Rhythm as a Master Regulator: The circadian rhythm, controlled by the suprachiasmatic nucleus (SCN), is a 24-hour cycle that influences sleep-wake patterns, hormone release, body temperature, digestion, and cognitive functions. Disruptions can lead to metabolic diseases like diabetes and cardiovascular issues.
Molecular Clocks and Hierarchical System: The SCN acts as the master clock, coordinating the peripheral clocks in organs like the liver, heart, and kidneys. These clocks align biological processes like energy use and hormone secretion with external cues like light, ensuring proper synchronization of bodily functions.
Importance of Circadian Amplitude: High circadian amplitude, representing strong oscillations between activity and rest, ensures optimal regulation of processes like metabolism and energy balance. Low amplitude leads to misalignment, increasing risks of chronic diseases such as diabetes and cardiovascular disease.
Phase-Specific Circadian Roles: The circadian rhythm operates in distinct phases, including the morning cortisol surge, daytime peak performance, and evening melatonin release. These phases help optimize energy usage, alertness, and cognitive function during the day, while supporting recovery and repair at night.
Key Factors Influencing Circadian Rhythm: Light exposure, consistent sleep-wake times, and regular meal timing are essential for maintaining a healthy circadian rhythm. Disruptions like late-night light exposure or irregular sleep can desynchronize the circadian clock, negatively impacting metabolic and cognitive functions.
AMPK and Circadian Rhythm Interaction: AMPK, an energy-sensing protein, plays a crucial role in synchronizing metabolic health with the circadian rhythm. It regulates processes like glucose metabolism and fat oxidation and influences core clock proteins like CRY1, linking metabolic status with circadian timing.
SIRT1's Role in Metabolism and Circadian Clock: SIRT1, a protein involved in metabolism and longevity, interacts with the circadian clock by regulating the BMAL1 protein and the mTOR pathway. This helps optimize energy usage and cellular repair during the day-night cycle, supporting metabolic health and aging
Chronotype and Circadian Misalignment: Chronotypes (early bird vs. night owl) and factors like social jetlag can disrupt the circadian clock, particularly for late chronotypes. This misalignment can lead to insulin resistance, inflammation, and increased risks for metabolic disorders like type 2 diabetes.
Blue and Red Light's Impact on Circadian Rhythm and Metabolic Health: Blue light, especially from electronic devices in the evening, disrupts the circadian rhythm by inhibiting melatonin production, delaying sleep onset, and impairing glucose metabolism. This can lead to increased insulin resistance, glucose levels, and a higher risk of metabolic disorders like type 2 diabetes. On the other hand, red light is less disruptive to melatonin production and circadian rhythms. Studies suggest that red light therapy can improve sleep quality, enhance glucose metabolism, and reduce insulin resistance by lowering oxidative stress and inflammation. Limiting blue light exposure at night and using red light may optimize circadian health and support metabolic function.
Citations
Green, C. B., Takahashi, J. S., & Bass, J. (2008). The meter of metabolism. Cell, 134(5), 728–742.
Loro, R. C., Azambuja, R., Silva, A., & Mezzomo, K. M. (2015). Low-level laser therapy in the treatment of type 2 diabetes mellitus: A pilot study. Lasers in Medical Science, 30(3), 861-866.
Latest Longevity Research Straight to your Inbox
Sign up for The Longevity Blueprint, a weekly newsletter from Healthspan analyzing the latest longevity research.
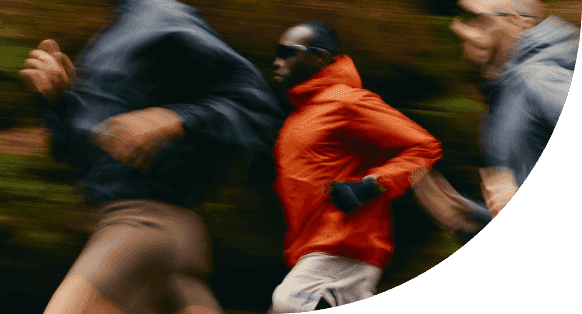
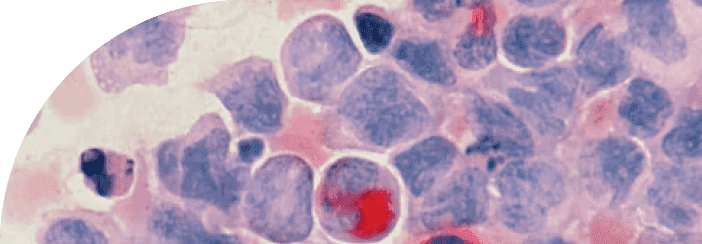

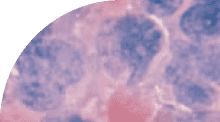