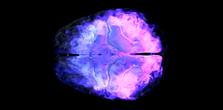
How Skeletal Muscle Mass Impacts Cognitive Health
Sarcopenia, commonly associated with the frailty of advanced age, goes beyond mere muscle weakness—it holds potential implications for our cognitive faculties. But what links the muscle's decline to the brain's? The answer, it seems, could lie in the realm of myokines—specific chemicals our muscles dispatch during exercise, intricately connected to brain health. Factor in disturbances like insulin imbalances, protein metabolism anomalies, compromised mitochondrial functions, mounting inflammation, and a portrait of cognitive erosion emerges. In their recent review, Oudbier et al. dissect these connections. In this research review, we dive into their analysis, revealing the biochemical and physiological connections between skeletal muscle and the brain, and the pathophysiological mechanisms underlying cognitive decline.
longevity
30 mins
By: Daniel Tawfik
The term 'sarcopenia' might evoke images of weakening muscles and reduced physicality in the elderly. But this decline in muscle mass and strength, prevalent among older adults, has ramifications far beyond just physical fragility. It's not just about the heightened risks of falls, fractures, or the increasing reliance on external care—sarcopenia might also be quietly chipping away at cognitive health.
Recent data shows a concerning trend: older adults with signs of sarcopenia are over three times more likely to have dementia compared to their peers without muscle loss. While weaker grip strength and reduced muscle mass are already established indicators of cognitive decline, the reasons behind this connection are still unknown.
But what could muscles possibly have to do with our brains?
The answer, it seems, could lie in the realm of myokines—specific chemicals our muscles dispatch during exercise, intricately connected to brain health. Factor in disturbances like insulin imbalances, protein metabolism anomalies, compromised mitochondrial functions—the very energy hubs of our cells—and mounting inflammation, and a portrait of cognitive erosion emerges.
In a recent comprehensive review titled Pathophysiological Mechanisms Explaining the Association Between Low Skeletal Muscle Mass and Cognitive Function, Oudbier et al. attempt to answer this question. The review draws data from over a hundred publications on associations between various measures of muscle strength and physical performance and cognition, the biochemical and physiological connections between skeletal muscle and the brain, and the pathophysiological mechanisms underlying cognitive decline.
Central to their findings are four pivotal mechanisms potentially bridging muscle deterioration with cognitive aging: the domino effects of systemic inflammation, insulin resistance, abnormal protein accumulation, and mitochondrial dysfunction.
The Aging Muscle: What's Really Happening Inside?
As we age, our muscles undergo a fascinating yet challenging transformation. By the time we're 60, we've already lost between 3% to 8% of our muscle mass every decade from the age of 30 (14).
But what's driving this loss?
Our muscles are composed of two main types of fibers: the slow-twitch type I fibers and the fast-twitch type II fibers. Think of type II fibers as the sprinters of the muscle world with their high energy bursts, while type I fibers are more like marathon runners, owing to their high mitochondrial content and endurance capacity (15). Intriguingly, as we age, it's the type II "sprinters" that disappear faster than the type I "marathoners" (16).
Our aging bodies also witness a decline in immune function, marked by a rise in pro-inflammatory agents like IL-1β, IL-6, and TNF-α (16). This inflammation sets off a cascade of events, from impaired mitochondria (our cell's powerhouses) that produce less energy to a surge in harmful reactive oxygen species (ROS) that further damage these mitochondria (16). This process leads to metabolic issues like insulin resistance and triggers the ubiquitin-proteasome system (UPS), a primary agent in muscle degradation (17).
At the heart of muscle shrinkage is a phenomenon called anabolic resistance. Imagine giving your muscles the building blocks they need to grow, but they just can't respond as effectively. This is largely because of a decline in the response to anabolic signals, like the availability of amino acids, in our older years (16). There's a typical signaling pathway, led by a player named mTOR, that should spring into action when these amino acids are present. But, as age catches up, mTOR doesn't respond as robustly (18). So, even if older individuals consume the same amount of protein as their younger counterparts, they don't synthesize it as efficiently (16).
To compound these challenges, the number of mitochondria diminishes with age, and these powerhouses also accumulate mutations in their DNA (19). This sets the stage for cell death. Plus, with increasing oxidative stress, ROS production surges, setting off a self-damaging cycle where faulty mitochondria lead to even more dysfunctional mitochondria (19).
In essence, our muscles are like an orchestra where each part plays a crucial role in the symphony of movement. As we age, some instruments start to falter, affecting the entire ensemble. In the following sections, we'll delve deeper into how this muscle "orchestra" connects with our cognitive abilities.
Aging Mind: Piecing Together the Puzzle of Cognitive Decline
Age is an inevitable factor we all grapple with, and it remains the leading risk factor not just for muscle mass loss but also for cognitive decline. To put things into perspective, as per the World Health Organization (WHO), a staggering 55 million individuals around the globe are grappling with dementia (20). What's more concerning? As our global population ages, we're looking at a potential doubling of dementia cases every five years (21).
But what exactly happens to our brains as we grow older?
The changes in our brains are intricate. Contrary to popular belief, we don't lose a massive number of neurons as we age. In fact, age-related neuronal loss in our central nervous system (CNS) is less than 10%, and this loss is confined to specific regions (23).
Instead, the shifts are more subtle but profound: we see fewer axons, the communication lines of our neurons, a reduction in the junctions (or synapses) where neurons communicate, shorter dendrites (the branches that reach out from neurons), and a loss of dendritic spines, tiny protrusions on dendrites that play a crucial role in transmitting information (22). These changes, many experts believe, are significant contributors to the cognitive slide associated with age (22,23).
Drawing the line from muscle mass loss to cognitive decline isn't straightforward. But certain molecular mechanisms offer compelling links between the two, such as changes in the secretion of muscle-specific chemicals (myokines), inflammation, insulin issues, protein accumulation anomalies, oxidative stress, and, not surprisingly, problems in our cellular powerhouses, the mitochondria (24).
One key player in this narrative is neuroinflammation. As we age, our body produces more of certain inflammatory chemicals. Now, while the brain is often seen as a fortress guarded by the blood-brain barrier (BBB), these chemicals can sometimes breach these defenses, leading to what we call neuroinflammation, or inflammation in the brain (24).
In conditions like Alzheimer's Disease (AD), it's evident how these processes can exacerbate disease progression. The accumulation of a protein called Aβ in AD patients, for example, triggers a kind of immune cell in the brain called microglia (24). Microglia are like the brain's janitors, cleaning up debris and maintaining order. However, with age, these microglia may not function as efficiently, producing more inflammatory chemicals instead (24).
Insulin is another potential root cause that gets dysregulated as we get older. While most of us associate insulin with blood sugar regulation, it also has a critical role in our brain.
Insulin that works in our brain is transported from our pancreas, and its receptors in the brain become fewer as we age (25,26). This might sound trivial, but a disruption in brain insulin dynamics can set the stage for neurodegenerative diseases. When insulin doesn't function optimally in the brain, it can lead to a cascade of problems, from increased concentrations of harmful proteins like tau to changes in brain plasticity and heightened inflammation, all contributing to cognitive issues (25,27).
Another pivotal area in understanding age-related cognitive decline involves the dysregulation of mTOR as we age. When it comes to our cells' ability to manage and use proteins, a key player emerges: mTOR. Think of mTOR as a master switch that governs how cells grow, divide, and manage their protein resources (18).
One of the challenges our cells face as we age is keeping a balanced protein "ecosystem"—making sure proteins are correctly produced, folded, and disposed of. This balance, termed proteostasis, is crucial to our cell's health. mTOR is central to this process, ensuring proteins are correctly synthesized and, when they're old or damaged, efficiently cleared away through processes like autophagy or degradation in cellular "recycling centers" called proteasomes.
However, mTOR's role is not confined to protein management and cell growth. It's also deeply involved in how our cellular powerhouses, the mitochondria, function. mTOR has a hand in the creation of new mitochondria and in regulating their dynamics and efficiency (18).
As we age, however, we know mTOR signaling can get disturbed. This disturbance has a domino effect, influencing not just how our mitochondria function but also how our brain manages its energy resources, glucose metabolism, and the cleanup of old or damaged cellular components (28).
Mitochondria dysfunction offers another potential mechanism to understand the relationship between cognitive decline and sarcopenia. As we age, mitochondrial damage accumulates. One of the culprits behind this damage is the accumulation of mutations in the mitochondria's own DNA, known as mtDNA. This happens largely due to rogue molecules called Reactive Oxygen Species (ROS), which can inflict molecular damage (29). Imagine these ROS as little sparks causing short circuits in our cellular machinery.
To make matters even more intricate, both inflammation and already-damaged mitochondria can further ramp up the production of these ROS molecules (29). This sets up a concerning feedback loop: impaired mitochondria produce more ROS, which in turn causes more damage to the mitochondria, further hindering their ability to produce energy for the cell (24,29). Understanding this cycle is crucial in our journey to decipher and potentially mitigate the effects of brain aging.
Mechanism 1: The Inflammatory Connection
Sarcopenia seems to be closely tied to a kind of low-level, systemic inflammation (30). This subtle inflammation is itself associated with cognitive impairment and even dementia (31). As we age, our body's immune system undergoes changes, a phenomenon dubbed "immunosenescence" (32). What does this mean? Simply put, our defenses against infections drop, resulting in a weakened immune response. Further complicating matters is another age-related issue called "inflamm-aging" (32), a state of lingering inflammation that doesn't resolve itself like typical inflammation would.
But Where Is This Inflammation Coming From?
A sedentary lifestyle might be playing a role. Older individuals who are less active have higher levels of inflammation-promoting substances from their fat tissues compared to their active counterparts (33, 34). For context, these inflammatory agents are notably higher in people with type 2 diabetes, particularly one called IL-6 (35, 36).
The Brain's Response: When Defense Turns Destructive
These inflammatory markers, circulating throughout the body, have been linked to an increased risk of dementia. Elevated markers like C-reactive protein (CRP) and IL-6 in the bloodstream are strong indicators of dementia risk (37). Both localized inflammation in the CNS and this systemic inflammation contribute to cognitive downturns (38).
While there is debate about whether the primary sources of cognitive decline are within the brain or come from the rest of the body, it's clear that there's a crucial two-way communication going on between the two.
They communicate with the brain through specialized receptors (39) and can stifle the birth of new neurons. Case in point: the inflammatory marker CCL11 increases with age and has been shown to hamper neurogenesis (40). However, there might be a silver lining. When scientists gave young mice an antibody against CCL11, the harmful effects on neurogenesis were reversed (40).
Lastly, and most concerning, these inflammatory agents seem to kick-start a cascade of events in the brain. Once these peripheral inflammatory agents make their way to the CNS, they can intensify localized inflammation. For instance, microglial cells, the brain's resident immune cells, can get activated to produce more local inflammatory agents like IL-1β and IL-6 (41,42).
This ongoing loop can meddle with normal brain functions, such as memory encoding, and exacerbate the damaging effects of toxic substances like Aβ (43, 44). This cycle of inflammation and damage could be a driving force behind the deterioration of our cognitive faculties.
The Role of Proinflammatory Cytokines in Brain Health: A Deep Dive into IL-6
While we often associate inflammation with visible problems, like swelling or redness after an injury, there's an equally important type of inflammation taking place inside our bodies on a microscopic level. A key player in this process is a cytokine called IL-6, and its role in the health of our central nervous system (CNS) is just starting to be understood.
IL-6: Not Just Any Cytokine
IL-6 is a bit of a paradox. Emitted by our skeletal muscle fibers during contraction, it can act as an anti-inflammatory agent (45). But this molecule is versatile, having both helpful and harmful effects on our CNS, depending on how it operates.
Signaling Mechanisms: Dual Pathways of IL-6
Diving a little deeper, IL-6 can send messages in two distinct ways within nervous tissues:
Classical Signaling: Here, IL-6 binds to a fixed receptor on the surface of certain cells like immune cells and myocytes (46).
Trans-signaling:A more complex route. In this pathway, IL-6 connects with a soluble version of its receptor (46). This interaction allows IL-6 to communicate with cells that don't have the fixed receptor, like neurons. The key player here is a protein called gp130, which lets IL-6 dock and then send its messages inside the cell.
IL-6 and Muscle Contraction
Interleukin-6 (IL-6) isn't just any cytokine; it holds a dual role. When secreted by type I and II skeletal muscle fibers during muscle contraction, it acts as an anti-inflammatory cytokine (45).
Interestingly, it's this trans-signaling route where IL-6 seems to show its harmful, pro-inflammatory side (47). Studies in mice have suggested that this pathway plays a role in the progression of several inflammatory diseases, like liver cancer and inflammatory bowel disease (48). This same mechanism might also be at play in the inflammation observed during CNS aging. Blocking trans-signaling can halt disease progression, pointing towards its critical role in inflammatory pathologies and inflamm-aging in the CNS (48,49).
A Dangerous Cocktail: Sedentary Behavior and Obesity
Here's where modern lifestyles come into the picture. Those who are obese tend to have higher levels of IL-6R and a specific enzyme, ADAM17 (50). This enzyme is responsible for creating that soluble IL-6 receptor we talked about. With this in mind, one could speculate that a sedentary lifestyle, characterized by inactive muscles and increased fat tissue, could amplify the harmful trans-signaling of IL-6. This enhanced signaling might drive "inflamm-aging" and, in turn, neurodegeneration. In this scenario, IL-6 produced from excess or inflamed fat tissue may bind to these soluble receptors, leading to harmful effects in our CNS (51).
Role of Myokines
Muscles in action do more than just contract; they function similarly to endocrine organs, releasing a range of cytokines and peptides termed "myokines" (52). As muscles undergo atrophy and witness the depletion of type II fibers in favor of type I fibers, the secretion patterns of myokines might alter.
A fascinating mix, myokines can either fan the flames of inflammation or dampen them. The array includes notable members like IL-6, IL-8, IL-15, and the brain-derived neurotrophic factor (BDNF) (52, 53, 54).
When muscles remain dormant and inactive, they tend to limit their endocrine capabilities. This shift leans towards promoting inflammation, which, in the long run, can escalate the risk of dementia (55). An interesting observation comes from a decade-long study from Dr. Mika Kivimaki's lab that pinpointed heightened systemic levels of IL-6 as potential harbingers of cognitive decline as people approach the later stages of midlife (56).
One crucial question that needs to be addressed is whether myokines can traverse the blood-brain barrier (BBB). Imagine our brain as a guarded fortress. One of its most formidable defenses is the blood-brain barrier (BBB), a highly selective semi-permeable border that prevents most substances in the circulating blood from non-selectively crossing over into the brain's fragile environment. The BBB is vital for protecting the brain from potential toxins and pathogens, but its very effectiveness presents a puzzle for researchers: How do certain beneficial molecules get through?
Myokines have the potential to relay the benefits of physical activity to the brain, potentially promoting neurogenesis (the growth of new neurons) and combating neurodegenerative diseases. However, for them to make a difference in brain health, they must first breach the formidable BBB.
While this remains an area of speculation, it's hard to ignore that certain cytokines have already showcased this ability through specific pathways in the brain's structure, specifically the circumventricular organs nestled close to the hypothalamus (57). These areas lack the typical BBB, making them possible gateways for certain substances to interact with the brain.
This opens up intriguing possibilities for other exercise-induced myokines like cathepsin B and FNDC5/irisin (58, 59). Recent research spotlights cathepsin B's impressive ability to cross the BBB. Once across, it appears to boost the brain's levels of neurotrophins—proteins that support neuron survival and growth. Among these, BDNF is especially significant, being a key player in brain plasticity and memory. (58).
Another pathway shines a light on exercise's role in elevating the expression of a gene and protein named peroxisome proliferator-activated receptor-gamma coactivator-1-alpha (PGC1α) in muscle cells. When we engage in physical activity, the expression of PGC1α in muscle cells is elevated. This molecule isn't just any ordinary protein; it acts as a central hub, mediating critical processes like oxidative phosphorylation and mitochondrial biogenesis. These are fancy terms for how our cells produce energy and how new cellular powerhouses, called mitochondria, are formed.
But PGC1α also kickstarts the expression of another protein, FNDC5. This protein then releases a peptide, a small chain of amino acids, named irisin into our bloodstream (58).
The current scientific theory is that once irisin is circulating in our blood, it can cross into the brain. And in this new territory, it potentially performs a remarkable feat: boosting the expression of BDNF, a protein integral to brain plasticity and memory formation. In essence, this could mean that the act of exercising might directly stimulate our brain's capacity to create new neurons and strengthen memory.
The value of BDNF isn't just theoretical. In real-world scenarios involving community-dwelling older women, a tangible link emerged between low plasma BDNF levels and diminished cognitive capabilities (60). Even more alarming, these lowered BDNF concentrations signaled a heightened risk of mortality over a subsequent five-year period (61). Delving into the realm of Alzheimer's Disease (AD), a stark contrast emerges: peripheral BDNF levels in AD patients trail significantly behind those of their non-AD counterparts (62).
Why might this be significant? Muscular contraction becomes a focal point. When muscles flex and release, they don't just move bones; they also spike BDNF mRNA and protein expression within muscle tissues, resulting in a surge of BDNF protein in the bloodstream (58).
Yet, the brain's central BDNF expression and function get curtailed when proinflammatory cytokine concentrations climb, taking a toll on neurogenesis (63). Interestingly, post-exercise BDNF in the bloodstream predominantly hails from the brain itself (52), suggesting a delicate balance or even a threshold of BDNF that must be maintained to effectively influence neurogenesis (64).
Levels of irisin are also integral to our understanding of the relationship between sarcopenia and cognitive decline. The speculation is that its levels in the bloodstream may directly influence its concentrations in the brain (65).
Examining AD models reveals a compelling story. Exercise's beneficial impacts on memory and synaptic plasticity get muted if there's a blockade of irisin, both in the periphery and the brain (65).
Delving further into the AD narrative, researchers have observed that levels of FNDC5/irisin dip in vital brain regions like the hippocampus and cerebrospinal fluid (CSF) when compared to their healthy peers or even those with just mild cognitive issues (65). But amidst these findings is a silver lining: a budding positive relationship between the concentrations of irisin and BDNF in the CSF (66), emphasizing the interconnectedness of these factors and their potential influence on cognitive health.
Mechanism 2: Insulin Metabolism
The central role of skeletal muscle in managing glucose homeostasis comes into focus when considering its major role in glucose storage and metabolism (67). Picture the skeletal muscle —not just as those structures that give our body shape or power our movements, but as intricate biochemical powerhouses. Their function goes beyond aiding physical movements; they play a pivotal role in the management and storage of glucose, the primary energy currency for our cells.
Every time we eat, our body breaks down carbohydrates into glucose, which then travels in our bloodstream to be used by our cells for energy. The skeletal muscle is like a reservoir, storing excess glucose and releasing it when needed, thus maintaining a delicate balance (67).
As skeletal muscle mass diminishes - a natural occurrence with age or due to certain conditions—the body's ability to manage glucose takes a hit. This reduction in muscle mass is not merely about decreased physical strength or mobility; it can lead to insulin resistance (68).
Insulin resistance has emerged as a standalone risk factor, potentially steering the ship towards cognitive decline (69). This suggests that our muscles and their role in glucose management are inextricably linked to our physical well-being and cognitive health.
A complex but detrimental cycle begins to emerge with aging; impaired glucose tolerance, a companion of insulin resistance, frequently appears as we grow older (70). This impaired tolerance has been linked with longitudinal changes in brain functionality, even in older individuals without any signs of cognitive dysfunction, affecting aspects such as regional cerebral blood flow (71).
It is critical to note that the brain primarily depends on glucose for its energy metabolism (72), establishing a direct pathway through which erratic glucose concentrations in peripheral tissues could hinder normal glucose regulation and insulin sensitivity in the brain, causing a cascade of complications (73).
As individuals age, the problem of insulin resistance continues to magnify (9). Insulin and glucose don't just operate in isolation; they cross the blood-brain barrier (BBB) to influence cerebral effects—remodeling synapses, steering neurotransmitter expression, and enhancing glucose metabolism in specific brain regions (74).
However, hyperinsulinemia, a condition where there's an excess of insulin in the blood, damages this system by reducing the number of insulin receptors in the BBB. Think of it as closing the gates to the brain, which then restricts the flow of insulin inside. The outcome? A potential cascade of harmful effects, including damage to the delicate blood vessels within the brain (74).
When our body continually swims in a pool of high glucose, it scrambles to compensate by pumping out more insulin, leading to hyperinsulinemia. Neurons, the very building blocks of our brain, suffer in this overflow (75).
Alzheimer's Disease (AD) provides a poignant example of how this glucose-insulin imbalance wreaks havoc on the brain. AD brings with it a pronounced disruption in how the brain uses and takes in glucose (75).
Here's the concerning cascade: The onset of peripheral insulin resistance can give rise to hyperinsulinemia. This doesn't just raise an alarm in the bloodstream; it disrupts insulin signaling right within the central nervous system. Like dominoes falling one after the other, this derangement sets off a series of adverse reactions.
The protein tau, often linked with neurodegenerative diseases, undergoes excessive phosphorylation—when tau is overly phosphorylated, it tends to aggregate, forming tangled bundles known as "tau tangles" or "neurofibrillary tangles." The presence of these tangles is believed to disrupt neuronal communication and might lead to cell death, contributing to the cognitive decline observed in AD and other tauopathies.
Oxidative stress, the silent cellular destroyer, escalates. And the infamous amyloid-beta (Aβ), a hallmark of AD, turns increasingly toxic. Together, these factors create a compounding negative effect, launching a multi-pronged attack on the brain and setting the stage for cognitive deterioration (75).
The brain's ability to regulate insulin and utilize it for various functions is pivotal for maintaining cognitive health. Interestingly, the movement of insulin across the blood-brain barrier (BBB) isn't simply a passive phenomenon.
Instead, it relies predominantly on an energy-driven saturable transport system (76). This system ensures that the brain has access to the right amount of insulin to meet its metabolic demands and execute functions tied to learning, memory, and cognition.
Chronic surges in peripheral insulin levels have been linked to notably diminished insulin concentrations in the brain (74). Now, why is this significant? Alzheimer's Disease, at its core, is deeply intertwined with the accumulation of amyloid-beta (Aβ) plaques. Like debris clogging a stream, these plaques accumulate and disrupt the brain's pathways. The twist in the tale is that this is closely tied to the brain's diminishing insulin levels. The shortage of insulin doesn't merely represent a figure; it obstructs the crucial process of Aβ clearance.
Are the deficits in brain insulin directly tied to the spike in peripheral insulin levels, especially those accentuated by a decline in skeletal muscle mass? Or could there be other factors, hidden threads we've yet to discover, that contribute to this delicate balance?
Alzheimer's Disease provides a compelling backdrop for this exploration. The myriad of mechanisms linking peripheral hyperinsulinemia to AD creates a maze of biological interactions. Within this maze, the intricate interaction between the insulin-degrading enzyme (IDE) and amyloid-beta (Aβ) stands out.
IDE is an enzyme that's responsible for degrading insulin once it has fulfilled its role. But beyond insulin, IDE is also known to degrade other small peptides, including Aβ.
Given that both insulin and Aβ can be substrates for IDE, there's a competition between them for degradation. If there's an excess of insulin (as in hyperinsulinemia), IDE may be more occupied with degrading insulin, leaving behind more Aβ. This can lead to an accumulation of Aβ in the brain, forming the plaques that are a hallmark of AD.
An increase in Aβ levels in the brain due to insufficient degradation can promote the formation of insoluble plaques. These plaques disrupt cellular function, potentially causing neuronal death and leading to the cognitive decline characteristic of AD. Furthermore, Aβ accumulation can also facilitate the formation of neurofibrillary tangles made up of tau protein.
Mechanism 3: Protein Metabolism, Muscle Mass, and Cognitive Decline
Central to our understanding of skeletal muscle dynamics is the balance between muscle protein synthesis (MPS) and muscle protein breakdown (MPB). With advancing age, the scales tilt, leaning towards what is termed "anabolic resistance" (82), where there's a decline in protein synthesis.
This encompasses a myriad of protein-related alterations, spanning from protein folding to its eventual breakdown (83). The cascading effects of this imbalance might reverberate beyond the muscular system, hinting at diminished protein concentrations in the brain, thus casting shadows on cognitive functions.
Contrary to what we might assume, misfolded and aggregated proteins aren't just accidental waste products our body churns out as errors. Instead, they bear the hallmark of several types of dementia, serving as microscopic red flags for the aging brain.
Think of them as old machinery parts that no longer fit where they should, accumulating over time. The heightened presence of such defective proteins is a testament to the challenges our body faces in protein degradation, especially as we age (84,85).
Yet, there's another player in this pathway—oxidative stress. Imagine oxidative stress as the rust that corrodes machinery, undermining its function. Oxidative stress doesn't just harm any component; it zeroes in on proteins essential for neurotransmission (how neurons communicate), proteostasis (maintaining cellular protein balance), and energy metabolism (how cells produce energy) (86). In aging brains, particularly those with Alzheimer's Disease (AD), this oxidative damage appears more severe (24). These aren't mere specks of rust; they're signs of machinery on the brink of collapse.
Lastly, the aggregation of misfolded proteins that become toxic to neuronal health extends to the relationship between the ubiquitin-dependent proteolytic system (UPS) and the Amyloid Precursor Protein (APP).
The ubiquitin-dependent proteolytic system (UPS) and the Amyloid Precursor Protein (APP) are two critical components in cellular processes, and their interplay has implications for neurodegenerative diseases, particularly Alzheimer's Disease (AD).
The UPS is a major cellular pathway for protein degradation. Proteins targeted for degradation are tagged with a small protein called ubiquitin, marking them for destruction by the proteasome, a protein complex responsible for disassembling unwanted proteins. Efficient function of the UPS ensures that misfolded or damaged proteins are rapidly removed, maintaining protein homeostasis in the cell.
APP is a transmembrane protein whose function is still not fully understood. However, it is best known for its role in AD. Specific proteolytic cleavage of APP can generate the peptide amyloid-beta (Aβ). In AD, Aβ peptides aggregate, forming insoluble plaques in the brain.
Any misstep in the function of the UPS during this interaction, or an overwhelming of its capacities, might trigger a build-up of amyloid-beta (Aβ), further driving the Disease's progression (87). Additionally, the ubiquitin—part and parcel of the UPS—has been found within the very Aβ deposits and neurofibrillary tangles, AD's infamous hallmarks, adding another dimension to this already complex tale (87).
Mechanism 4: Mitochondrial Dysfunction
The mitochondrion is often described as the powerhouse of the cell, pivotal for skeletal muscle energetics. Within its intricate matrix, a number of vital processes occur—from oxidative phosphorylation driving ATP synthesis, regulating apoptosis, to controlling ROS production (89). Like the many gears in a machine, when one is misaligned, the system risks dysfunction.
Reactive oxygen and nitrogen species (RONS) play essential roles in cellular processes, especially within muscle tissues. Under normal circumstances, RONS are involved in various signaling processes that help guide molecular events (17). However, when these species are produced in excessive amounts, they lead to oxidative stress, resulting in damage to cells and their structures (90).
This process of oxidative stress has been identified as a common mechanism underlying two distinct age-related conditions: sarcopenia, which involves a decrease in skeletal muscle mass and function, and dementia, which encompasses various cognitive impairments.
The "oxidative stress theory" suggests that an accumulation of RONS is a primary factor driving cellular aging, or senescence (90). Within the scope of sarcopenia, mitochondrial abnormalities have been found to play a significant role in muscle loss (17). Similarly, in dementia, disruptions in mitochondrial function are strongly associated with cognitive deficits (91,92). An ongoing area of research is exploring whether changes in the mitochondria of peripheral tissues, such as muscles, can influence mitochondrial function in the brain.
Decline in Muscle Energy Production
A notable marker of aging is the decline in skeletal muscle's ability to undergo oxidative phosphorylation, a crucial process by which cells generate energy (19,93). Accompanying this decline are accumulating deletions in mitochondrial DNA (mtDNA). These genetic changes aren't just passive alterations; they actively push forward oxidative damage within tissues (19).
The Domino Effect: Cellular Life and Death
Cellular apoptosis, often viewed as a programmed cell death mechanism, becomes more pronounced in this environment. When our mitochondria—the cellular powerhouses—lose their efficiency, the frequency of this programmed death increases (94). The ripple effect of mitochondrial inefficiency triggers a series of reactions. It not only stalls the growth and division of cells but also initiates a state called the senescence-associated secretory phenotype, creating a highly inflammatory environment (95).
There's a theory, known as the "mitochondrial cascade hypothesis," which offers a map to navigate the complex connections between age-related decline in mitochondrial function and certain brain changes linked to Alzheimer's Disease (102). In simple terms, this hypothesis shines light on how problems with our mitochondria may lead to loss of synaptic connections in the brain, the creation of Aβ, tau protein changes, and the development of brain plaques.
Here's how it might work: as neurons, our brain cells, use up their energy supplies to produce Aβ, they eventually reach a point where they just can't keep up. The outcome? A drop in the number of mitochondria and, interestingly, a decrease in Aβ production as well (103).
From a medical standpoint, this theory underscores an alarming fact: when the symptoms of Alzheimer's Disease finally become apparent, the build-up of amyloid in the brain has already reached its peak. In other words, the Disease might have been progressing quietly for a long time before showing outward signs (104). It highlights the pressing need for early detection and intervention in Alzheimer's Disease.
The mitochondria, with their multifaceted roles, stand at the nexus of sarcopenia and dementia. As scientific research forges ahead, the aim is to decipher this multifaceted relationship and target interventions for optimal cellular health.
Interplay Between Inflammation, Insulin, Protein Metabolism, and Mitochondrial Function; Myokines: Muscle-Derived Peptides Orchestrating Interorgan Crosstalk
Myokines are emerging as potential key links in understanding the intricate machinery that underpins cognitive decline. Envisioning them as conductors in an orchestra, these peptides can modulate various instruments: inflammation, insulin signaling, protein metabolism, and mitochondrial function, orchestrating a symphony that determines the fate of cognitive health.
The relationship between skeletal muscle and cognitive health is not a simple one-way street. It's a bidirectional dialogue, with myokines at the heart of this conversation. Upon physical exertion, the muscle releases specific myokines that can engage with molecules in the brain, fostering neurogenesis, promoting nervous system development, and ensuring neuroprotection (7). For instance, FNDC5, by releasing its derivative peptide irisin, not only promotes neuronal growth but also marshals defense mechanisms in the brain (105). Similarly, voluntary physical activity has shown to regulate IL-6 levels in the hippocampus and temper systemic inflammatory response (106).
One key player in this narrative is BDNF. Not only is it involved in sparking mitochondrial health by optimizing fatty acid oxidation but also it amplifies PGC-1α, a cornerstone in mitochondrial biogenesis and myokine release like FDNC5/Irisin and cathepsin B (107,108). Importantly, the synthesis and release of these myokines are linked to physical activity. As a corollary, a sedentary lifestyle diminishes myokine release, paving the way for pro-inflammatory processes (109).
Furthermore, Cathepsin B, another significant myokine, oversees cell death signaling in mitochondria (110). As age marches on, the body witnesses a decline in motor units, diminishing the inventory of muscle fibers associated with peripheral axons (111). This decline, potentially exacerbated by limited physical activity, could influence the very mechanisms we've highlighted - inflammation, insulin response, and mitochondrial health. The recent identification of neurturin, another myokine, further underscores this relationship. Its rise in skeletal muscles post-exercise hints at its role in fine-tuning the neuromuscular junction (112).
It's important to emphasize that the relationships highlighted here represent only a fraction of the vast interplay between these physiological pathways. Many pieces of this jigsaw puzzle remain, and myokines represent just a few of those pieces. But their potential as arbiters in the complex symphony of muscle-brain crosstalk is becoming increasingly evident.
In essence, understanding how these myokines interact with each of the physiological mechanisms can pave the way for interventions that might slow or halt cognitive decline. Encouraging physical activity might be one of the most potent ways to ensure this orchestra plays a harmonious tune.
The Two-Way Street: Does the Brain Influence Muscle as Much as Muscle Influences the Brain?
While much attention has been placed on how muscle health can affect cognitive function, the inverse relationship is equally intriguing and less explored: can cognitive health, or lack thereof, impact muscle health?
A significant portion of the studies cited in Oudbier's review adopt a cross-sectional approach, capturing a snapshot of the relationship between muscle and cognition at a single point in time. However, these snapshots may not accurately represent the dynamic, evolving relationship between the brain and muscle. There's a pressing need for longitudinal studies, which observe and measure these variables over extended periods, to understand the evolving dance between muscle health and cognitive function.
The relationship is unlikely to be a simple one-directional path where only the muscle influences the brain. There's mounting evidence suggesting this relationship could be bidirectional. For instance, while low muscle mass and strength have been associated with cognitive impairment, the reverse also seems true: cognitive impairment can lead to decreased muscle mass and strength (113–115).
This idea of a reciprocal relationship introduces a concerning possibility: a vicious cycle where cognitive decline accelerates muscle loss, which in turn further exacerbates cognitive impairment. This cyclical relationship, if confirmed, paints a picture of intertwined fates, where the decline in one domain could trigger a cascade of declines in the other.
In conclusion, the relationship between muscles and the brain is intricate and complex. It's essential to consider the possibility of a two-way street when understanding how these two systems interact. As research progresses, it's critical to break free from linear models and appreciate the multi-dimensional dance between cognition and muscle health. Future research should aim to elucidate this potential feedback loop and its implications for interventions and treatments.
Citations
Cruz-Jentoft AJ, Bahat G, Bauer J, et al. Sarcopenia: revised European consensus on definition and diagnosis. Age Ageing. 2019;48(1):16–31. doi:10.1093/ageing/afy169
Yeung SS, Reijnierse EM, Pham VK, et al. Sarcopenia and its association with falls and fractures in older adults: a systematic review and meta‐analysis. J Cachexia Sarcopenia Muscle. 2019;10(3):485–500. doi:10.1002/jcsm.12411
Wang DX, Yao J, Zirek Y, Reijnierse EM, Maier AB. Muscle mass, strength, and physical performance predicting activities of daily living: a meta‐analysis. J Cachexia Sarcopenia Muscle. 2020;11(1):3–25. doi:10.1002/jcsm.12502
Pacifico J, Geerlings MA, Reijnierse EM, Phassouliotis C, Lim WK, Maier AB. Prevalence of sarcopenia as a comorbid disease: a systematic review and meta-analysis. Exp Gerontol. 2020;131:1–19. doi:10.1016/j.exger.2019.110801
Cipolli GC, Yassuda MS, Aprahamian I. Sarcopenia is associated with cognitive impairment in older adults: a systematic review and meta-analysis. J Nutr Health Aging. 2019;23(6):525–531. doi:10.1007/s12603-019-1188-8
Beeri MS, Leugrans SE, Delbono O, Bennett DA, Buchman AS. Sarcopenia is associated with incident Alzheimer’s dementia, mild cognitive impairment, and cognitive decline. J Am Geriatr Soc. 2021;182:6–1835. doi:10.1111/jgs.17206
Chen W, Wang L, You W, Shan T. Myokines mediate the cross talk between skeletal muscle and other organs. J Cell Physiol. 2021;239:3–2412. doi:10.1002/jcp.30033
Severinsen MCK, Pedersen BK. Muscle–organ crosstalk: the emerging roles of myokines. Endocr Rev. 2020;41(4):594–609. doi:10.1210/endrev/bnaa016
Cleasby ME, Jamieson PM, Atherton PJ. Insulin resistance and sarcopenia: mechanistic links between common co-morbidities. J Endocrinol. 2016;229(2):R67–R81. doi:10.1530/JOE-15-0533
Wu H, Jang J, Dridi S, et al. Net protein balance correlates with expression of autophagy, mitochondrial biogenesis, and fat metabolism‐related genes in skeletal muscle from older adults. Physiol Rep. 2020;8(19):e14575. doi:10.14814/phy2.14575
Ferri E, Marzetti E, Calvani R, Picca A, Cesari M, Arosio B. Role of age-related mitochondrial dysfunction in sarcopenia. Int J Mol Sci. 2020;21(15):5236. doi:10.3390/ijms21155236
Tuttle CSL, Thang LAN, Maier AB. Markers of inflammation and their association with muscle strength and mass: a systematic review and meta-analysis. Ageing Res Rev. 2020;64:101185. doi:10.1016/j.arr.2020.101185
Kwan P. Sarcopenia, a neurogenic syndrome? J Aging Res. 2013;2013:791679. doi:10.1155/2013/791679
Volpi E, Nazemi R, Fujita S. Muscle tissue changes with aging. Curr Opin Clin Nutr Metab Care. 2004;7(4):405–410. doi:10.1097/01.mco.0000134362.76653.b2
Yakabe M, Ogawa S, Akishita M. Clinical manifestations and pathophysiology of sarcopenia. RNA Transcr. 2015;1(2):10–17. doi:10.11648/j.rnat.20150102.11
Nishikawa H, Fukunishi S, Asai A, Yokohama K, Nishiguchi S, Higuchi K. Pathophysiology and mechanisms of primary sarcopenia. Int J Mol Med. 2021;48(2):1–8. doi:10.3892/ijmm.2021.4989
Picca A, Calvani R, Bossola M, et al. Update on mitochondria and muscle aging: all wrong roads lead to sarcopenia. Biol Chem. 2018;399(5):421–436. doi:10.1515/hsz-2017-0331
Papadopoli D, Boulay K, Kazak L, et al. mTOR as a central regulator of lifespan and aging. F1000Res. 2019;8:9981–9921. doi:10.12688/f1000research.17196.1
Peterson CM, Johannsen DL, Ravussin E. Skeletal muscle mitochondria and aging: a review. J Aging Res. 2012;2012. doi:10.1155/2012/194821
Fact Sheet Dementia. World Health Organization.
https://www.who.int/news-room/fact-sheets/detail/dementia
. Updated September 2, 2021. Accessed February 5, 2022.
Cao Q, Tan C-C, Xu W, et al. The prevalence of dementia: a systematic review and meta-analysis. J Alzheimers Dis. 2020;73(3):1157–1166. doi:10.3233/JAD-191092
Murman DL. The impact of age on cognition. Semin Hear. 2015;36(3):111–121. doi:10.1055/s-0035-1555115
Pannese E. Morphological changes in nerve cells during normal aging. Brain Struct Funct. 2011;216(2):85–89. doi:10.1007/s00429-011-0308-y
Poddar J, Pradhan M, Ganguly G, Chakrabarti S. Biochemical deficits and cognitive decline in brain aging: Intervention by dietary supplements. J Chem Neuroanat. 2019;95:70–80. doi:10.1016/j.jchemneu.2018.04.002
Ma L, Wang J, Li Y. Insulin resistance and cognitive dysfunction. Clin Chim Acta. 2015;444:18–23. doi:10.1016/j.cca.2015.01.027
Kullmann S, Heni M, Hallschmid M, Fritsche A, Preissl H, Häring H-U. Brain insulin resistance at the crossroads of metabolic and cognitive disorders in humans. Physiol Rev. 2016;96(4):1169–1209. doi:10.1152/physrev.00032.2015
Biessels GJ, Reagan LP. Hippocampal insulin resistance and cognitive dysfunction. Nat Rev Neurosci. 2015;16(11):660–671. doi:10.1038/nrn4019
Perluigi M, Di Domenico F, Butterfield DA. mTOR signaling in aging and neurodegeneration: at the crossroad between metabolism dysfunction and impairment of autophagy. Neurobiol Dis. 2015;84:39–49. doi:10.1016/j.nbd.2015.03.014
Chakrabarti S, Munshi S, Banerjee K, Thakurta IG, Sinha M, Bagh MB. Mitochondrial dysfunction during brain aging: role of oxidative stress and modulation by antioxidant supplementation. Aging Dis. 2011;2(3):242–256.
Tuttle CS, Waaijer ME, Slee‐Valentijn MS, Stijnen T, Westendorp R, Maier AB. Cellular senescence and chronological age in various human tissues: a systematic review and meta‐analysis. Aging Cell. 2020;19(2):e13083. doi:10.1111/acel.13083
Koyama A, O’Brien J, Weuve J, Blacker D, Metti AL, Yaffe K. The role of peripheral inflammatory markers in dementia and Alzheimer’s disease: a meta-analysis. J Gerontol A Biol Sci Med Sci. 2013;68(4):433–440. doi:10.1093/gerona/gls187
Fulop T, Larbi A, Pawelec G, et al. Immunology of aging: the birth of inflammaging. Clin Rev Allergy Immunol. 2021:1–14. doi:10.1007/s12016-021-08899-6
Ramsey KA, Rojer AG, D’Andrea L, et al. The association of objectively measured physical activity and sedentary behavior with skeletal muscle strength and muscle power in older adults: a systematic review and meta-analysis. Ageing Res Rev. 2021;67:101266. doi:10.1016/j.arr.2021.101266
Rezuş E, Burlui A, Cardoneanu A, et al. Inactivity and skeletal muscle metabolism: a vicious cycle in old age. Int J Mol Sci. 2020;21(2):592. doi:10.3390/ijms21020592
Akbari M, Hassan-Zadeh V. IL-6 signalling pathways and the development of type 2 diabetes. Inflammo Pharmacol. 2018;26(3):685–698. doi:10.1007/s10787-018-0458-0
Sadeghabadi ZA, Abbasalipourkabir R, Mohseni R, Ziamajidi N. Investigation of oxidative stress markers and antioxidant enzymes activity in newly diagnosed type 2 diabetes patients and healthy subjects, association with IL-6 level. J Diabetes Metab Disord. 2019;18(2):437–443. doi:10.1007/s40200-019-00437-8
Darweesh SK, Wolters FJ, Ikram MA, de Wolf F, Bos D, Hofman A. Inflammatory markers and the risk of dementia and Alzheimer’s disease: a meta-analysis. Alzheimer’s Dement. 2018;14(11):1450–1459. doi:10.1016/j.jalz.2018.02.014
Shen X-N, Niu L-D, Wang Y-J, et al. Inflammatory markers in Alzheimer’s disease and mild cognitive impairment: a meta-analysis and systematic review of 170 studies. J Neurol Neurosurg Psychiatry. 2019;90(5):590–598. doi:10.1136/jnnp-2018-319148
Krabbe KS, Pedersen M, Bruunsgaard H. Inflammatory mediators in the elderly. Exp Gerontol. 2004;39(5):687–699. doi:10.1016/j.exger.2004.01.009
Villeda SA, Luo J, Mosher KI, et al. The ageing systemic milieu negatively regulates neurogenesis and cognitive function. Nature. 2011;477(7362):90–94. doi:10.1038/nature10357
Ferreira ST, Clarke JR, Bomfim TR, De Felice FG. Inflammation, defective insulin signaling, and neuronal dysfunction in Alzheimer’s disease. Alzheimer’s Dement. 2014;10(1):S76–S83. doi:10.1016/j.jalz.2013.12.010
Dilger RN, Johnson RW. Aging, microglial cell priming, and the discordant central inflammatory response to signals from the peripheral immune system. J Leukoc Biol. 2008;84(4):932–939. doi:10.1189/jlb.0208108
Maher F, Nolan Y, Lynch MA. Downregulation of IL-4-induced signalling in hippocampus contributes to deficits in LTP in the aged rat. Neurobiol Aging. 2005;26(5):717–728. doi:10.1016/j.neurobiolaging.2004.07.002
Blasko I, Grubeck-Loebenstein B. Role of the immune system in the pathogenesis, prevention and treatment of Alzheimer’s disease. Drugs Aging. 2003;20(2):101–113. doi:10.2165/00002512-200320020-00002
Pedersen BK, Febbraio M. Muscle-derived interleukin-6—a possible link between skeletal muscle, adipose tissue, liver, and brain. Brain Behav Immun. 2005;19(5):371–376. doi:10.1016/j.bbi.2005.04.008
Schumertl T, Lokau J, Rose-John S, Garbers C. Function and proteolytic generation of the soluble interleukin-6 receptor in health and disease. Biochim Biophys Acta Mol Cell Res. 2022;1869(1):119143. doi:10.1016/j.bbamcr.2021.119143
Rose-John S. Therapeutic targeting of IL-6 trans-signaling. Cytokine. 2021;144:155577. doi:10.1016/j.cyto.2021.155577
Garbers C, Heink S, Korn T, Rose-John S. Interleukin-6: designing specific therapeutics for a complex cytokine. Nat Rev Drug Discovery. 2018;17(6):395–412. doi:10.1038/nrd.2018.45
Burton MD, Sparkman NL, Johnson RW. Inhibition of interleukin-6 trans-signaling in the brain facilitates recovery from lipopolysaccharide-induced sickness behavior. J Neuroinflammation. 2011;8(1):1–13. doi:10.1186/1742-2094-8-54
Kraakman MJ, Kammoun HL, Allen TL, et al. Blocking IL-6 trans-signaling prevents high-fat diet-induced adipose tissue macrophage recruitment but does not improve insulin resistance. Cell Metab. 2015;21(3):403–416. doi:10.1016/j.cmet.2015.02.006
Campbell IL, Erta M, Lim SL, et al. Trans-signaling is a dominant mechanism for the pathogenic actions of interleukin-6 in the brain. J Neurosci. 2014;34(7):2503–2513. doi:10.1523/JNEUROSCI.2830-13.2014
Pedersen BK. Muscles and their myokines. J Exp Biol. 2011 2011;214:337–346. doi:10.1242/jeb.048074
Brandt C, Pedersen BK. The role of exercise-induced myokines in muscle homeostasis and the defense against chronic diseases. Biomed Res Int. 2010;2010:1–6. doi:10.1155/2010/520258
Matthews VB, Åström M-B, Chan M, et al. Brain-derived neurotrophic factor is produced by skeletal muscle cells in response to contraction and enhances fat oxidation via activation of AMP-activated protein kinase. Diabetologia. 2009;52(7):1409–1418. doi:10.1007/s00125-009-1364-1
Pedersen BK. Exercise-induced myokines and their role in chronic diseases. Brain Behav Immun. 2011;25(5):811–816. doi:10.1016/j.bbi.2011.02.010
Singh-Manoux A, Dugravot A, Brunner E, et al. Interleukin-6 and C-reactive protein as predictors of cognitive decline in late midlife. Neurology. 2014;83(6):486–493. doi:10.1212/WNL.0000000000000665
Komaki G, Arimura A, Koves K. Effect of intravenous injection of IL-1 beta on PGE2 levels in several brain areas as determined by microdialysis. Am J Physiol Endocrinol Metab. 1992;262(2):E246–E251. doi:10.1152/ajpendo.1992.262.2.e246
Pedersen BK. Physical activity and muscle–brain crosstalk. Nat Rev Endocrinol. 2019;15(7):383–392. doi:10.1038/s41574-019-0174-x
Moon HY, Becke A, Berron D, et al. Running-induced systemic cathepsin B secretion is associated with memory function. Cell Metab. 2016;24(2):332–340. doi:10.1016/j.cmet.2016.05.025
Komulainen P, Pedersen M, Hänninen T, et al. BDNF is a novel marker of cognitive function in ageing women: the DR’s EXTRA Study. Neurobiol Learn Mem. 2008;90(4):596–603. doi:10.1016/j.nlm.2008.07.014
Krabbe KS, Mortensen EL, Avlund K, et al. Brain‐derived neurotrophic factor predicts mortality risk in older women. J Am Geriatr Soc. 2009;57(8):1447–1452. doi:10.1111/j.1532-5415.2009.02345.x
Xie B, Zhou H, Liu W, et al. Evaluation of the diagnostic value of peripheral BDNF levels for Alzheimer’s disease and mild cognitive impairment: results of a meta-analysis. Int J Neurosci. 2020;130(3):218–230. doi:10.1080/00207454.2019.1667794
Calabrese F, Rossetti AC, Racagni G, Gass P, Riva MA, Molteni R. Brain-derived neurotrophic factor: a bridge between inflammation and neuroplasticity. Front Cell Neurosci. 2014;8:430. doi:10.3389/fncel.2014.00430
Liu PZ, Nusslock R. Exercise-mediated neurogenesis in the hippocampus via BDNF. Front Neurosci. 2018;12:1–6. doi:10.3389/fnins.2018.00052
Lourenco MV, Frozza RL, de Freitas GB, et al. Exercise-linked FNDC5/irisin rescues synaptic plasticity and memory defects in Alzheimer’s models. Nat Med. 2019;25(1):165–175. doi:10.1038/s41591-018-0275-4
Lourenco MV, Ribeiro FC, Sudo FK, et al. Cerebrospinal fluid irisin correlates with amyloid‐β, BDNF, and cognition in Alzheimer’s disease. Alzheimer’s Dement. 2020;12(1):e12034. doi:10.1002/dad2.12034
Sylow L, Tokarz VL, Richter EA, Klip A. The many actions of insulin in skeletal muscle, the paramount tissue determining glycemia. Cell Metab. 2021;33(4):758–780. doi:10.1016/j.cmet.2021.03.020
Kim K, Park SM. Association of muscle mass and fat mass with insulin resistance and the prevalence of metabolic syndrome in Korean adults: a cross-sectional study. Sci Rep. 2018;8(1):1–8. doi:10.1038/s41598-018-21168-5
Ekblad LL, Rinne JO, Puukka P, et al. Insulin resistance predicts cognitive decline: an 11-year follow-up of a nationally representative adult population sample. Diabetes Care. 2017;40(6):751–758. doi:10.2337/dc16-2001
Chang AM, Halter JB. Aging and insulin secretion. Am J Physiol Endocrinol Metab. 2003;284(1):E7–E12. doi:10.1152/ajpendo.00366.2002 Google ScholarPubMedWorldCat
Thambisetty M, Beason-Held LL, An Y, et al. Impaired glucose tolerance in midlife and longitudinal changes in brain function during aging. Neurobiol Aging. 2013;34(10):2271–2276. doi:10.1016/j.neurobiolaging.2013.03.025
Mergenthaler P, Lindauer U, Dienel GA, Meisel A. Sugar for the brain: the role of glucose in physiological and pathological brain function. Trends Neurosci. 2013;36(10):587–597. doi:10.1016/j.tins.2013.07.001
Roh E, Kim M-S. Emerging role of the brain in the homeostatic regulation of energy and glucose metabolism. Exp Mol Med. 2016;48(3):e216–e216. doi:10.1038/emm.2016.4
Cholerton B, Baker LD, Craft S. Insulin, cognition, and dementia. Eur J Pharmacol. 2013;719(1-3):170–179. doi:10.1016/j.ejphar.2013.08.008
Nguyen TT, Ta QTH, Nguyen TTD, Le TT. Role of insulin resistance in the Alzheimer’s Disease progression. Neurochem Res. 2020;45(7):1481–1491. doi:10.1007/s11064-020-03031-0
Rhea EM, Banks WA. A historical perspective on the interactions of insulin at the blood‐brain barrier. J Neuroendocrinol. 2021;33(4):e12929. doi:10.1111/jne.12929 Google ScholarPubMedWorldCat
Barclay RD, Burd NA, Tyler C, Tillin NA, Mackenzie RW. The role of the IGF-1 signaling cascade in muscle protein synthesis and anabolic resistance in aging skeletal muscle. Front Nutr. 2019;6:146. doi:10.3389/fnut.2019.00146
Jarmusch S, Baber L, Bidlingmaier M, et al. Influence of IGF-I serum concentration on muscular regeneration capacity in patients with sarcopenia. BMC Musculoskelet Disord. 2021;22(1):1–11. doi:10.1186/s12891-021-04699-3
Bian A, Ma Y, Zhou X, et al. Association between sarcopenia and levels of growth hormone and insulin-like growth factor-1 in the elderly. BMC Musculoskelet Disord. 2020;21(1):1–9. doi:10.1186/s12891-020-03236-y
Wennberg AM, Hagen CE, Machulda MM, et al. The association between peripheral total IGF-1, IGFBP-3, and IGF-1/IGFBP-3 and functional and cognitive outcomes in the Mayo Clinic Study of Aging. Neurobiol Aging. 2018;66:68–74. doi:10.1016/j.neurobiolaging.2017.11.017
Kim IY, Park S, Jang J, Wolfe RR. Understanding muscle protein dynamics: technical considerations for advancing sarcopenia research. Ann Geriatr Med Res. Sep 2020;24(3):157–165. doi:10.4235/agmr.20.0041
Burd NA, Gorissen SH, Van Loon LJ. Anabolic resistance of muscle protein synthesis with aging. Exerc Sport Sci Rev. 2013;41(3):169–173. doi:10.1097/JES.0b013e318292f3d5
Anisimova AS, Alexandrov AI, Makarova NE, Gladyshev VN, Dmitriev SE. Protein synthesis and quality control in aging. Aging (Albany NY). 2018;10(12):4269. doi:10.18632/aging.101721
Götz J, Ittner LM. Animal models of Alzheimer’s disease and frontotemporal dementia. Nat Rev Neurosci. 2008;9(7):532–544. doi:10.1038/nrn2420
Lovestone S, McLoughlin D. Protein aggregates and dementia: is there a common toxicity?. J Neurol Neurosurg Psychiatry. 2002;72(2):152–161. doi:10.1136/jnnp.72.2.152
Domínguez M, de Oliveira E, Odena MA, Portero M, Pamplona R, Ferrer I. Redox proteomic profiling of neuroketal-adducted proteins in human brain: regional vulnerability at middle age increases in the elderly. Free Radic Biol Med. 2016;95:1–15. doi:10.1016/j.freeradbiomed.2016.02.034
Al Mamun A, Uddin MS, Kabir MT, et al. Exploring the promise of targeting ubiquitin-proteasome system to combat Alzheimer’s disease. Neurotox Res. 2020;38(1):8–17. doi:10.1007/s12640-020-00185-1
Sakuma K, Yamaguchi A. Recent advances in pharmacological, hormonal, and nutritional intervention for sarcopenia. Pflugers Arch. 2018;470(3):449–460. doi:10.1007/s00424-017-2077-9
Gan Z, Fu T, Kelly DP, Vega RB. Skeletal muscle mitochondrial remodeling in exercise and diseases. Cell Res. 2018;28(10):969–980. doi:10.1038/s41422-018-0078-7
Liguori I, Russo G, Curcio F, et al. Oxidative stress, aging, and diseases. Clin Interv Aging. 2018;13:757–772. doi:10.2147/CIA.S158513
Tobore TO. On the central role of mitochondria dysfunction and oxidative stress in Alzheimer’s disease. Neurol Sci. 2019;40(8):1–14. doi:10.1007/s10072-019-03863-x
Khacho M, Harris R, Slack RS. Mitochondria as central regulators of neural stem cell fate and cognitive function. Nat Rev Neurosci. 2019;20(1):34–48. doi:10.1038/s41583-018-0091-3
McKenzie D, Bua E, McKiernan S, Cao Z, Wanagat J, Aiken JM. Mitochondrial DNA deletion mutations: a causal role in sarcopenia. Eur J Biochem. 2002;269(8):2010–2015. doi:10.1046/j.1432-1033.2002.02867.x
Marzetti E, Calvani R, Cesari M, et al. Mitochondrial dysfunction and sarcopenia of aging: from signaling pathways to clinical trials. Int J Biochem cell Biol. 2013;45(10):2288–2301. doi:10.1016/j.biocel.2013.06.024
Chapman J, Fielder E, Passos JF. Mitochondrial dysfunction and cell senescence: deciphering a complex relationship. FEBS Lett. 2019;593(13):1566–1579. doi:10.1002/1873-3468.13498
White AR, Du T, Laughton KM, et al. Degradation of the Alzheimer disease amyloid β-peptide by metal-dependent up-regulation of metalloprotease activity. J Biol Chem. 2006;281(26):17670–17680. doi:10.1074/jbc.M602487200
Limoge M, Safina A, Beattie A, Kapus L, Truskinovsky AM, Bakin AV. Tumor-fibroblast interactions stimulate tumor vascularization by enhancing cytokine-driven production of MMP9 by tumor cells. Oncotarget. 2017;8(22):35592–35608. doi:10.18632/oncotarget.16022
Rocha M, Hernandez-Mijares A, Garcia-Malpartida K, Banuls C, Bellod L, Victor V M. Mitochondria-targeted antioxidant peptides. Curr Pharm Des. 2010;16(28):3124–3131. doi:10.2174/138161210793292519
Turrens JF. Mitochondrial formation of reactive oxygen species. J Physiol. 2003;552(2):335–344. doi:10.1113/jphysiol.2003.049478
Leuner K, Schütt T, Kurz C, et al. Mitochondrion-derived reactive oxygen species lead to enhanced amyloid beta formation. Antioxid Redox Signal. 2012;16(12):1421–1433. doi:10.1089/ars.2011.4173
Wong KY, Roy J, Fung ML, Heng BC, Zhang C, Lim LW. Relationships between mitochondrial dysfunction and neurotransmission failure in Alzheimer’s disease. Aging Dis. 2020;11(5):1291–1316. doi:10.14336/AD.2019.1125
Swerdlow RH. Mitochondria and mitochondrial cascades in Alzheimer’s disease. J Alzheimers Dis. 2018;62(3):1403–1416. doi:10.3233/JAD-170585
Swerdlow RH, Burns JM, Khan SM. The Alzheimer’s disease mitochondrial cascade hypothesis: progress and perspectives. Biochim Biophys Acta Mol Basis Dis. 2014;1842(8):1219–1231. doi:10.1016/j.bbadis.2013.09.010
Ismail R, Parbo P, Madsen LS, et al. The relationships between neuroinflammation, beta-amyloid and tau deposition in Alzheimer’s disease: a longitudinal PET study. J Neuroinflammation. 2020;17:1–11. doi:10.1186/s12974-020-
Latest Longevity Research Straight to your Inbox
Sign up for The Longevity Blueprint, a weekly newsletter from Healthspan analyzing the latest longevity research.
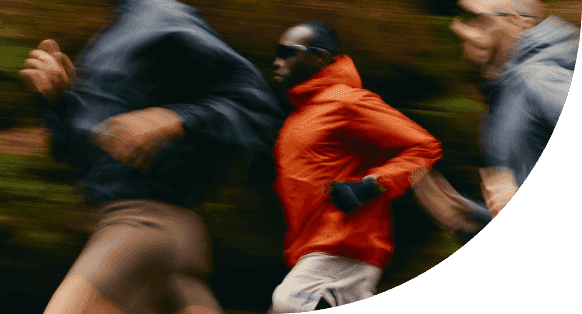
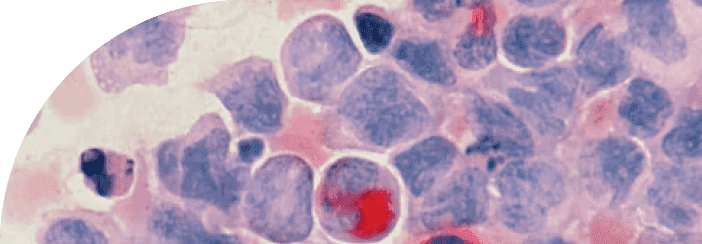

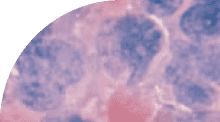